1 John M. Cullen and Matthew Breen North Carolina State University, USA Cancer is a disease of the genome, arising from DNA alterations that dysregulate gene structure or function.1,2 Damage to the cellular genome or altered expression of genes is a common feature for virtually all neoplasms.3 Given that there is an inherent error rate in DNA replication, all multicellular organisms face the near certainty of developing a neoplasm if they survive long enough, as essential mutations for neoplastic transformation will eventually develop. Many mutations may be inconsequential, but cancer can develop when nonlethal mutations occur in a small subset of the coding and noncoding regions of the genome, perhaps affecting even just a few of the ~20,000 genes thought to comprise the mammalian genome.4 There are many agents, in addition to deficiencies in DNA replication fidelity and error repair, that drive tumor formation. These agents include viruses, mutagenic chemicals, and radiation. Unraveling the pathogenesis of cancer has not only helped us understand how a cell transforms into a tumor but has also promoted molecular tests that now help diagnose and provide prognoses for a variety of cancers in humans and animals. Genetic damage is a universal component of the pathogenesis of neoplasia, with somatic mutations in genes identified in 90% of cases, and germ line mutations identified in 20% of human neoplasia and both features found in a small percentage of neoplasms.1,2,4 In some cases a single base pair mutation is sufficient to alter a critical amino acid, leading to altered protein function and an increased risk for neoplastic transformation. Other types of mutations involve insertions, deletions, or duplications of gene segments. Structural chromosomal changes, such as translocations, which lead to chimeric transcripts or deregulation of gene expression through movement of promoters and enhancer regions adjacent to relevant genes can also drive malignant transformation. In addition, gene copy number increases or decreases (gene dosage) can also occur. DNA sequence mutations are not the only route to neoplasia.5 Epigenetic mechanisms regulate gene expression without causing structural changes to the genome and also play a role in malignant transformation.6 Epigenetic changes are reversible, heritable alterations of gene expression without mutation of the genome. Three main forms of epigenetic gene regulation include DNA methylation, histone acetylation, and microRNA expression. Gene expression can be silenced, diminished, or increased by altering methylation patterns in the DNA. Aberrant methylation patterns, such as hypermethylation and hypomethylation, are common in a variety of neoplasms and are linked to abnormal gene expression levels. In particular, methylation of tumor suppressor genes leading to their suppression is recognized in a number of human cancers, including breast, colon, and renal carcinomas. Histone proteins serve as spools that are wound with DNA strands to package cellular DNA into nucleosomes, which when compacted constitute the main components of chromatin. Gene expression can be altered by loosening or tightening the binding of the DNA strands to the histone proteins. Tightly wound DNA is either not transcribed or transcribed at lower levels than when it is more loosely associated with the histone proteins. Histone proteins that have been altered, often by acetylation, have a more relaxed binding pattern with their associated DNA and this facilitates gene expression. MicroRNAs (miRNAs) are small, nontranscribed RNA molecules, approximately 22 nucleotides in length, that contribute to a complex termed the RNA‐silencing complex, which binds to specific sequences in messenger RNA strands and directs gene silencing.7 MiRNAs may regulate up to 30% of genes via posttranscriptional control. Amplifications and deletions of miRNAs are common in various human cancers and more work is needed to evaluate animal neoplasms.8 Consequently, miRNAs can participate in tumor formation, as increased expression of miRNAs that target tumor suppressor genes leads to an increased tumor risk, as does decreased expression of miRNAs that target oncogenes. Although none of these mechanisms alter the structure of the cellular genome they can significantly alter gene expression and have all been shown to be involved in neoplastic transformation.8 A combination of mutation and epigenetic mechanisms is involved in the multistep process that leads to the emergence of a population of cells with a malignant phenotype known as malignant transformation. Epigenetic perturbations may offer an explanation for some types of tumors associated with chronic inflammation or the presence of foreign material but no clear pattern of mutation, such as the fibrosarcomas and osteosarcomas that can develop in dogs infected with Spirocirca lupi or rare reports of sarcomas forming following metallic implants. Injection site sarcomas in cats seem to be associated with the inflammation induced by the vaccine adjuvant, although a subset of the affected cats have mutations in the tumor suppressor gene p53.9–11 There are many pathways that can lead an individual cell to the malignant phenotype, but they all involve multiple genetic and epigenetic alterations. Tumor progression is a process by which cells that have developed a neoplastic phenotype acquire more characteristics that lead to malignancy and metastasis. Tumor growth starts clonally with a single cell that has undergone neoplastic transformation and the incipient tumor develops by clonal expansion of that cell. When a population of cells is identified as clonal it is a strong indicator that the population is neoplastic. However, this is not universally true, as clonal lymphocyte populations can be identified in some inflammatory conditions such as cases of feline infectious peritonitis and infections with Ehrlichia sp. Initially, all cells in the neoplastic mass are identical, but due to the genetic instability the tumor cells acquire genetic and epigenetic changes that give rise to tumor heterogeneity (Figure 1.1).12 In some instances this genetic change can be dramatic and sudden, as seen in chromothripsis, a phenomenon in which hundreds to thousands of genetic rearrangements can occur in one or a few chromosomes during a single event. Processes frequently affected include various types of DNA repair, telomere maintenance, DNA replication, and chromosome segregation. Some genetic changes are lethal to the affected cells, whereas others confer new functions and phenotypes, giving inherent growth advantages. Over time, the developing tumor mass becomes composed of a heterogeneous cell population and the tumor accumulates characteristics that make them more dangerous to the host.12 As a neoplastic cell replicates, subclones emerge that are more locally aggressive, more likely to metastasize, and less responsive to therapy. Tumor progression has been attributed to a greater level of genomic instability in affected cells, which explains why early detection of the neoplasm is associated with improved prognosis. However, early detection is challenging in most clinical settings. By the time most malignant neoplasms are detected, using contemporary imaging methods, they most likely comprise a heterogeneous cell population, the neoplasms having completed the greater part of their growth. A single transformed cell must undergo at least 30 doublings to form a 1 g mass, an approximate cut‐off for clinical detection, but only approximately 10 more doublings are needed to form a 1 kg mass (Figure 1.2). Since a 1 kg mass is regarded as a lethal tumor burden for a human, it is likely that fewer doublings are needed to form lethal cancers in small domestic animals. Figure 1.1 Tumor cell heterogeneity. Although tumors arise from a single cell, the inherent genetic instability in tumor cells gives rise to additional mutations and a heterogeneous population of cells with different genetic characteristics. Some mutations are lethal to developing cell lines and they die, but other mutations provide various features that facilitate the emergence of viable cell lines which may contain malignant characteristics including the ability to metastasize. Figure 1.2 Tumor doubling. Tumor growth starts with a single cell that expands clonally. It takes approximately 30 doublings to form a 1 g mass, at which time most lesions can be detected clinically. Only 10 more doublings are needed to form a 1 kg mass, considered to be a lethal burden in humans. Likely, a smaller mass would be lethal in dogs or cats. Cancer is a multistep process and in some types of epithelial cancers there is a histologic phenotype that is characteristic of the different steps, including hyperplasia, dysplasia, and adenoma and carcinoma formation (Figure 1.3). Progressive accumulation of various mutations and epigenetic disturbances accompany these different histologic phenotypes. However, in some circumstances activation of an oncogene in an otherwise normal cell can lead to cell senescence and inhibit tumor formation.13 Paradoxically, expression of an activated oncogene can lead to an exit from the cell cycle and termination of cell growth. Oncogene‐induced senescence is consequently considered an authentic tumor suppressor mechanism in vivo. Ultimately, genetic and epigenetic alterations lead to a common pattern of features, or hallmarks, that distinguish neoplastic cell populations from normal cells. These hallmarks of cancer were initially proposed in 200014 and an updated review has been recently published.15 Each of these hallmarks and their relevance regarding animal carcinogenesis, diagnosis, prognosis and therapy will be discussed in this chapter. Figure 1.3 Histologic evolution of a carcinoma. The cellular development of cancer is a multistep process in most cases. There are several phenotypic steps in the evolution of colonic carcinoma including areas of hyperproliferation/hyperplasia, then dysplasia, followed by adenoma and, in a subset of these, carcinomas. The distribution of different phenotypes is not uniform throughout individual lesions and regions with different phenotypes may be seen when a lesion is sampled. Spontaneous growth arrest or resolution of tumors may occur, as overexpression of oncogenes can drive cellular senescence in some circumstances. The six key elements of malignancies are shown in Figure 1.4. Figure 1.4 Hallmarks of cancer. These features are key elements of malignancies. Normal tissues are often capable of responding to injury or tissue loss by proliferation. Proliferation is driven by growth factors that bind specific cellular receptors, often tyrosine kinases, causing them to become activated and propagating a cascade of intracellular signals that culminate in mitosis. However, proliferation is controlled and limited, retaining normal structure and function. In cancer, proliferation is persistent and unregulated. Cell proliferation and maturation are regulated by a subset of cellular genes. Proto‐oncogenes are normal genes that encode proteins participating in one or more signal transduction pathways associated with important regulatory pathways.16 Because of their central role in the life cycle of the cell, proto‐oncogenes have been conserved throughout evolution and their DNA sequences vary little from yeast to humans. Disturbances in gene structure or expression can alter the cellular function of a proto‐oncogene, causing it to stimulate tumor formation. Once this alteration has occurred, the proto‐oncogene is termed an oncogene. More than 100 oncogenes have been identified, and their numbers increase with continued genetic analyses of neoplasms. Typically the genes are referred to using a three‐letter nomenclature. Many oncogenes were initially identified as part of the genome of retroviruses that caused cancer and they were named for the virus from which they were originally identified. For example, the proto‐oncogene myc was originally isolated from the avian myelocytomatosis virus, and ERB A and ERB B were isolated from avian erythroblastosis virus. It should be remembered that it is not the oncogene, but the encoded protein that leads to cell transformation. The proteins encoded by oncogenes are referred to as oncoproteins. Oncogenes can be grouped into five categories based on the types of oncoproteins they encode. These categories include growth factors, growth factor receptors, intracellular signal transducers, nuclear regulatory proteins (transcription factors), and cyclins. The proto‐oncogene sis encodes the beta chain of platelet‐derived growth factor (PDGF). When fibroblasts are infected with simian sarcoma virus, a retrovirus that contains the oncogene sis, there is an excess of sis oncoprotein produced. This protein leads to overstimulation of PDGF receptors on the cell surface in an autocrine fashion and can drive fibroblasts towards malignant transformation. In this circumstance the oncoprotein has a normal amino acid sequence but is produced in an abnormal, deregulated amount. Mutant forms of growth factors also occur, and they may inappropriately stimulate receptors by binding to them in an abnormal fashion. Oncogenes may encode growth factor receptors. A typical growth factor receptor has three components: an extracellular growth factor binding domain, a transmembranous segment, and a cytoplasmic domain with kinase activity. Oncogene‐encoded growth factor receptors, such as ERB B, are often truncated into a form that no longer has the extracellular receptor portion of the normal protein. These abnormal receptors do not require growth factor binding to be stimulated and are constitutively activated. The intracellular signal transducers are located in the cytosol (e.g., ABL, RAF) or are membrane associated (e.g., RAS, SRC). Typically, these molecules are enzymes in the tyrosine kinase family. Point mutations or more gross structural alterations can constitutively activate these proteins, producing a level of activity that in turn leads to uncontrolled cell proliferation. Tyrosine kinase receptor activity is abnormal in several animal cancers, including mammary carcinomas and mast cell tumors, and several inhibitors of tyrosine kinase activity are being investigated, or are currently in use, as therapy.17 Transcription factors are nuclear proteins that regulate gene expression. They bind to selected sites on DNA in a complex with other proteins to facilitate gene expression. The oncoproteins encoded by MYC, JUN, and FOS are transcription factors that stimulate expression of genes necessary for cell division. Abnormal levels of expression, or mutations that alter the function of these proteins, can compromise growth control. Cyclins are a series of proteins that precisely regulate movement through the cell cycle.18 Individual cyclins are expressed for brief intervals at appropriate points in the cell cycle. The cyclins interact with and activate enzymes termed cyclin‐dependent kinases (CDKs). The CDKs, in turn, activate proteins that are essential for progression through the cell cycle. Disruption in the function of cyclins leads to dysregulated control of cell replication. Several types of tumors in humans have been described with mutations in the genes that encode cyclins or CDKs.19 Abnormal cyclin expression has been documented in canine and feline neoplasms, including cyclin A in mammary carcinomas and cyclin D1 in mammary tumors, squamous cell carcinomas, and, to a lesser extent, basal cell tumors.20,21 Proto‐oncogenes can be transformed into oncogenes following damage to their structure. Structural alterations can occur by mutation of individual nucleotides or alterations that may occur during changes to karyotype organization, such as chromosomal translocation events. Damage to individual nucleotides (i.e., point mutation) is the most common mutation sustained by proto‐oncogenes. Chemical carcinogens and some forms of radiation exert their influence this way. Mutation of a single nucleotide can lead to the incorporation of a novel amino acid into a protein, and, if appropriately localized, the activity of the protein can be profoundly altered. One of the better characterized signal transduction pathways affected by mutation involves the RAS (derived from rat sarcoma virus) signaling pathway (Figure 1.5). All mammalian cells express three related RAS proteins, designated K‐RAS, N‐RAS, and H‐RAS. Each of these proteins has a similar function, acting as an enzyme (GTPase) that phosphorylates GTP and acts as a switch regulating cell proliferation and survival. Any RAS family member can drive tumor development when they are mutated in specific codons. Figure 1.5 RAS oncogene. An example of proto‐oncogene activation is shown in this overview diagram of the typical RAS signaling cascade. When a growth factor binds to its transmembrane receptor the receptor becomes activated. Receptor binding triggers activation of RAS via a bridging protein. Inactive RAS, which is bound to guanosine diphosphate (GDP), becomes activated via an exchange (red arrow) for guanosine triphosphate (GTP). Activated RAS acts through intermediary proteins to activate mitogen‐activated protein kinases (MAP kinases) that lead to altered nuclear signal transduction and cell mitosis. In normal cells GTPase‐activating protein (GAP) stimulates dephosphorylation of activated RAS to an inactive form that curtails signaling (blue arrow). Mutant RAS does not interact with GAP normally and consequently stimulates cell proliferation in an unchecked fashion. Signaling via RAS begins when growth factors bind to specific cell surface receptors. This induces the receptors to dimerize, autophosphorylate, and undergo a conformational change. As a result of the conformational change, the receptors can interact with an associated bridging protein complex, which in turn transfers activation to the RAS protein located on the cytoplasmic surface of the cell membrane. Normally, the RAS protein is inactive and is bound to guanine diphosphate (GDP). When the RAS protein is stimulated it exchanges GDP for guanine triphosphate (GTP) and becomes activated. RAS protein is negatively regulated by GTPase‐activating protein (GAP), a protein that enhances the hydrolysis of RAS‐bound GTP to GDP. Activated RAS attracts a serine/threonine kinase, termed RAF (derived from rapidly accelerated fibrosarcoma), to the inner aspect of the cell membrane, where RAF is phosphorylated by membrane‐associated kinases. Activated RAF in turn phosphorylates mitogen‐activated protein (MAP) kinases, and these kinases migrate to the nucleus, where they stimulate the synthesis of nuclear transcription factors, such as MYC. These transcription factors stimulate the expression of genes that cause resting cells either to enter the cell cycle and divide or to alter their differentiation or synthesis patterns. Control of cell signaling is fine‐tuned by the balance of a matrix of stimulatory and inhibitory influences. Consequently, cell proliferation can be driven not only by stimulatory events, but also by the disruption of inhibitory pathways. The RAS gene offers a good example of this abnormality as well, as mutations in RAS typically impair the ability of the GAP protein to dephosphorylate RAS, causing it to remain in the active state, leading to a constitutive activation of RAS driving cell proliferation. Mutations in RAS are common in human tumors. In some surveys 16–30% of all tumors are reported to bear RAS mutations.22 Specific sites of human tumors bearing RAS mutations in 20% or more cases include the biliary tree, large intestine, small intestine, pancreas, and the skin. Evaluation of specific RAS isoforms reveals that they selectively appear in different tumor types.23 Specific isoforms can be quite common in individual tumor types. Mutations in K‐RAS are found in 22% of all human tumors with an incidence of 8% for N‐RAS and 3% for H‐RAS. N‐RAS is mutated in human melanoma, acute myelogenous leukemia, thyroid neoplasia, and multiple myeloma. K‐RAS, involved in pancreatic, colorectal, thyroid, and lung carcinomas and acute myelogenous leukemia among others, is the most frequently mutated RAS gene in human neoplasms. Approximately 61–90% of human pancreatic carcinomas contain a mutation in K‐RAS. Mutations in RAS are not nearly as well documented in animals as humans, as most veterinary studies are limited by small sample sizes, but RAS mutations are reported to be less frequent in a variety of sarcomas of dogs and cats than they are in humans.24–32 RAS mutations have been noted in several tumor types, including K‐RAS mutations in approximately 15–25% canine lung tumors of different types33–35 and N‐RAS mutations in canine leukemia, where up to 25% of cases of acute myeloid leukemia or lymphoid leukemia have mutations.36,37 Altered RAS signaling is one pathway among several alterations in signal transduction that lead to cellular hyperproliferation. The ability of malignant cells to proliferate in a sustained fashion can also derive from the impact of an excess of growth factors. Mitogenic signals can be generated in an autocrine fashion in circumstances where malignant cells release growth factors that bind their own receptors and initiate signaling that leads to proliferation. In a more complicated paracrine fashion, malignant cells can signal nearby stromal cells, causing them to release mitogenic factors that in turn stimulate the tumor cells. In some cases, mutations lead to multiple copies of growth factor receptors on an individual cell, leading to excessive intracellular signaling in the face of a normal amount of growth factors. Alternatively, malignant cells may become independent of growth factors when they have mutated growth factor receptors or signal transducers such as RAF that are constitutively activated stimulating the downstream signaling cascade. Another more clinically relevant example of abnormal proliferation caused by a mutation is found in canine and feline mast cell tumors. The proto‐oncogene c‐KIT (also referred to as CD117 and stem cell factor receptor) encodes the transmembrane tyrosine kinase receptor KIT. Following binding of its ligand, stem cell factor, the resulting signal transduction is involved in survival, maturation, migration, and proliferation of mast cells and other hematopoietic cells. Mutations in the c‐KIT gene, in particular small internal tandem repeats in exons 11 and 12, lead to an abnormal receptor that does need ligand binding as a prerequisite for activation, and consequently it constitutively stimulates signal transduction.38 There are several other mutations that also can activate c‐KIT.39 Abnormal localization of the gene product in the cytoplasm in neoplastic mast cells is also associated with increased proliferation of the affected cells and likely a poorer prognosis for dogs bearing these genetic alterations than those without such changes.40 The presence of internal tandem repeats (ITRs) can be detected by a PCR‐based assay, which identifies neoplastic mast cells in approximately 30% of malignant canine cutaneous mast cell tumors.41 Identification of the c‐KIT ITR mutations can be used for diagnostic purposes, to assess prognosis and to monitor response to therapy. Not all malignant mast cell tumors bear this mutation so its role is not entirely clear. However, targeting the tyrosine kinase activity of c‐KIT is recognized as a useful approach in a variety of human and veterinary applications. There are new chemotherapeutic agents that inhibit the tyrosine kinase activity of c‐KIT now available in veterinary medicine, Palladia® (Zoetis) and Kinavet® (AB Science) that are used to treat cutaneous mast cell tumors in dogs as well as other malignancies with aberrant c‐KIT activity. Chromosome translocation results in the movement of one chromosome to another chromosome, or exchange of segments between different chromosomes in reciprocal translocation events. This process can deregulate transcription by bringing in close juxtaposition active cellular promoters and proto‐oncogenes. One example occurs in both humans and mice: the proto‐oncogene MYC is overexpressed in lymphomas of B‐cell lineage due to translocation of an active cellular promoter from the immunoglobulin gene to another chromosome that contains MYC. In some circumstances the functions of proto‐oncogenes are altered by chromosome translocation. A well‐characterized example of this process occurs in the distinctive translocation that produces the Philadelphia chromosome found in up to 95% of cases of human chronic myelogenous leukemia (CML), but it is not specific, as it is found in approximately 25% of cases of human acute lymphoblastic leukemia and rarely in acute myelogenous leukemia.42 This rearrangement involves an exchange of chromosomal segments between the distal ends of human chromosomes 9q and 22q, resulting in a derivative chromosome 22 in which a fragment of the proto‐oncogene (abl) on human chromosome 9 becomes juxtaposed to the breakpoint cluster region (bcr) on human chromosome 22. This fusion yields an abnormal hybrid gene that encodes a chimeric messenger RNA containing information from both genes. When the message is translated, a hybrid protein, termed a fusion protein, results. In this circumstance the fusion protein is an active oncoprotein that results in elevated tyrosine kinase activity, which is crucial to its oncogenic potential. To halt progression of the leukemia the BCR‐ABL kinase antagonist imatinib mesylate (Gleevec®) is used in therapy. As a competitive inhibitor of the tyrosine kinase activity, the drug serves to block the proliferative signal given by the BCR‐ABL protein, preventing the formation of new abnormal cells. While this effect does not apply to all patients with CML it is sufficiently effective that Gleevec therapy is now considered standard of care for patients with the Philadelphia chromosome. Although CML is rare in domestic dogs, numerous cases have been shown to present with evolutionarily conserved cytogenetic change (structural chromosomal changes) resembling the Philadelphia translocation in human cases. In the canine aberration, termed the “Raleigh” chromosome, the canine genes BCR (dog chromosome 9) and ABL (dog chromosome 26) are juxtaposed and produce a fusion protein43,44 (Figure 1.6). These data suggest that treatment with Gleevec or other tyrosine kinase inhibitors could be an option for therapy of BCR‐ABL‐positive canine cases. The Raleigh chromosome has since been identified in additional canine leukemias, including chronic monocytic and acute myeloblastic cases.43,45 More study will be needed to determine the frequency of this translocation in canine CML and other leukemias. Cytogenetic analysis has been used to monitor response to therapy and revealed a marked reduction of circulating neoplastic cells with the translocation following therapy (vincristine and prednisolone) in one case of canine chronic monomyelocytic leukemia.46 Figure 1.6 Raleigh chromosome. In human leukemias, a characteristic chromosome is the Philadelphia chromosome (Ph). This derivative chromosome, also referred to as the Philadelphia translocation, is the result of reciprocal translocation between human chromosomes 9 and 22, bringing the genes BCR and ABL (panel A) together to create activation of the tyrosine kinase of c‐ABL. The evolutionarily conserved translocation (panel B) has been detected in canine leukemias, the result of a reciprocal translocation between regions of dog chromosomes 9 and 26 (shown in panel C). The canine event is referred to as the Raleigh chromosome and has been detected in chronic myelogenous leukemia and chronic myelomonocytic leukemia. Within these patients, the frequency of cells with the Raleigh chromosome has been shown to decrease in response to tyrosine kinase inhibitor treatment, indicating that its presence may be used to monitor cytogenetic remission. Gene expression can be altered via gene amplification or deletion, promoter insertion, gene translocation, and regulatory miRNA. Each of these genetic mechanisms can lead to the deregulated synthesis of normal (i.e., wild type) proto‐oncogene proteins. Given that many proteins encoded by proto‐oncogenes function to stimulate cell proliferation, it is obvious that their overexpression would have the potential to lead to cancer formation. For reasons that are not well understood, tumor cells often sustain excessive rounds of localized DNA replication that can result in the formation of multiple copies (hence the term gene amplification) of the same gene or genes. The duplicated genes (or amplicon) may be found in small chromosome‐like structures termed double minutes, or may form concatenated (i.e., like beads on a string) structures within a chromosome that can be identified as homogeneously staining regions (HSRs). HSRs are portions of chromosomes that lack the characteristic banding pattern found in normal chromosomes. In general, gene amplification leads to the overproduction of the products encoded by the genes within the amplicon, increasing the potential for neoplastic transformation. An additional and relatively new mechanism for influencing gene expression involves miRNA.47 There are more than 1000 types of miRNA expressed in essentially all cells. Primarily they bind to message RNA and promote degradation of messenger RNA, thereby preventing translation and influencing gene expression. Specific miRNAs are associated with some human neoplasms, particularly colorectal cancer and chronic lymphocytic leukemia, but more work is needed to evaluate the role of miRNAs in neoplasia of veterinary interest. In veterinary medicine there are several important oncogenic retroviruses. These include feline leukemia virus, bovine leukemia virus, and avian leukosis virus. When certain oncogenic retroviruses, known as nonacute retroviruses, insert their genome into cellular DNA, the regulatory elements normally controlling viral gene expression also affect the expression of nearby cellular host genes. Viruses and cells have two major types of these regulatory elements, enhancers and promoters. Both elements stimulate gene expression, but differ in their functional attributes. Promoters stimulate adjacent genes but must be properly oriented (upstream of the gene) to facilitate expression. Enhancers stimulate promoter activity, but unlike promoters, their capacity to stimulate transcription is orientation independent. Since, in general viral promoters and enhancers are more potent than their cellular counterparts, they can significantly increase and thus dysregulate cellular gene expression. When a retrovirus integrates within a region of genomic DNA flanking a proto‐oncogene, transcription of the proto‐oncogene can be deregulated, leading to cell transformation. In most circumstances, viral insertion events affect the regulation of gene expression, not the function of the gene or genes affected. There is also a second type of oncogenic retrovirus, called acute transforming retroviruses, that are typically replication defective, but carry an oncogene derived from a host’s proto‐oncogene in their genome and rapidly transform infected cells. Feline sarcoma virus is an example of this type of virus. Oncogenic DNA viruses generally differ from oncogenic retroviruses in that they contain authentic viral genes that encode oncoproteins capable of transforming infected cells. These viral proteins often act by interfering with the proteins encoded by tumor suppressor genes. Bovine papillomavirus and several primate herpes viruses are examples of oncogenic DNA viruses of veterinary importance. The phenotypic manifestation of the first hallmark of cancer (Sustaining Proliferative Signaling) is typically recognized as an increase in mitotic rate. Many tumor types have an increased rate of mitosis, enumerated by counting the number of mitotic figures observed in a specific number of high‐power (40 × objective) microscopic fields (the mitotic count in 2.37 mm2; see pp. 944–945) although there are several other immunohistochemical methods to assess cell proliferation, including staining for proliferating cell nuclear antigen (PCNA) or Ki67 that may be more readily interpreted than counting mitoses. The presence of an increased number of mitoses is considered in the diagnosis of benign or malignant forms of various tumors. For example, the mitotic count of soft tissue sarcoma in dogs is currently used as one of the principal criteria in the assessment of malignancy and to predict the likelihood of recurrence and or metastasis. However, increased mitoses alone are not necessarily an indication of malignancy since the tissue of origin requires consideration. For example, canine histocytomas are characterized by a high mitotic count yet these neoplasms typically regress spontaneously. A similar observation can be made for transmissible venereal tumors of dogs. There also are examples of tumors with a low proliferation index that behave aggressively, such as maxillary fibrosarcoma in dogs. In some cases, a high mitotic count may not correlate with the cellular proliferation of a mass. This occurs when there is arrest of mitosis, leaving elevated numbers of mitotic figures at a given point in time, but in the absence of completed cell division, no increase or a limited increase in cell population. A second hallmark of cancer involves the ability to bypass potent growth inhibitory signaling.48 The major agents of growth inhibition are a group of 25 or more tumor suppressor genes. Tumor suppressor genes play a critical role in the control of normal cell growth. They serve as the “brakes” to cell replication. When tumor suppressor genes are inactivated, cells lose regulatory control of cell proliferation. A single, intact, functional copy of a tumor suppressor gene is sufficient to maintain control of cell proliferation. When both alleles are lost or damaged the affected cell has a high risk of neoplastic transformation. The discovery of tumor suppressor genes arose from the study of certain human families that presented with a significantly increased incidence of specific tumor types. Genetic analysis of these “cancer families” revealed that some family members were born with one mutated allele of a critical gene, and when a second mutation in the functional allele occurred spontaneously the affected individual was at a very high risk to develop neoplasia.9 The first tumor suppressor gene to be discovered this way was the retinoblastoma or RB gene. Loss of both alleles led to the development of retinoblastomas in affected children. Loss of function of both alleles of another tumor suppressor gene, TP53, was identified in other kindreds and termed Li–Fraumeni syndrome. These individuals are at elevated risk for a variety of mesenchymal neoplasms, but mutated TP53 is frequently identified in many human malignancies.49,50 At least one heritable cancer syndrome (renal carcinoma and nodular dermatofibrosis, or RCND, of German shepherd dogs) has been described in dogs with an autosomal dominant inheritance.51,52 The heritable factor for this syndrome maps to dog chromosome 5 (CFA 5), and specifically to the tumor suppressor gene folliculin (FLCN, previously BDH gene). This region in the dog chromosome overlaps a corresponding region in the human chromosome that was recently described as the heritable factor for the corresponding human disease (Birt–Hogg–Dubé syndrome). Inactivation of this tumor suppressor gene is critical to the development of this syndrome. It is probable that other comparable syndromes to those that are described in humans will eventually be identified in companion and laboratory animals, but it is unlikely these will account for more than 5–10% of all cancers in animal cases. There are a few examples in dogs in which a specific cancer (melanoma, histiocytic sarcoma) is associated with the absence or decrease of tumor suppressor gene(s). It is likely there are inherited susceptibilities to cancer in many breeds. Examples are lymphoma in the golden retriever53 and parathyroid neoplasia in keeshonds.54 Ongoing cytogenetic studies are being conducted to assess the genetic basis of breed‐related alterations in tumor risk. To understand the relevance of tumor suppressor gene inactivation in tumorigenesis, a brief review of the normal cell cycle and how it differs from that in neoplastic cells is warranted. The cell cycle consists of a series of biochemically distinct temporal periods that prepare the cell for division.55 Following mitosis, a cell may withdraw from the cell cycle and enter a quiescent stage (G0 phase) or continue to proliferate. In most instances, cells in G0 can be recruited into the cell cycle when necessary by interactions with one or more growth factors. The first growth phase of the cell cycle is termed G1, for the gap in time between mitosis and the next round of DNA synthesis. The duration of this phase of the cell cycle is more variable than the duration of other phases, ranging from 6 to 12 hours. During G1, RNA and proteins are synthesized but no DNA is formed. Synthesis of DNA occurs in the S phase, during which the DNA content of the cell increases from diploid to tetraploid. The duration of the S phase is similar in all cells and takes from 3 to 8 hours. The S phase is followed by the G2 phase, a pause of about 3–4 hours that precedes mitosis. During the G2 phase the cell has two complete sets of diploid chromosomes. Mitosis, or the M phase, takes no more than an hour to complete in normal cells. The ability of cells to restrict or slow their movement through the cell cycle is regulated. This can be observed when normal cells in tissue culture are damaged, for example in irradiation‐induced genetic damage.56 Irradiated cells in the early stages of the cell cycle respond by halting their progress prior to the S phase; this pause in the cell cycle has been termed the G1/S checkpoint. During the pause, DNA that has been damaged by irradiation can be repaired before mutations are passed on to the genomes of daughter cells. In cells in which tumor suppressor genes are absent or not functioning properly, genetic damage is left unrepaired, which often leads to genetic instability in the daughter cells and additional oncogenic events. A similar checkpoint is present at the transition between the G2 and M phases of the cell cycle. The best characterized of the tumor suppressor genes are TP53 gene, activated only in cases of genetic damage or hypoxia, and the retinoblastoma (RB) gene, which is constitutively involved in the cell cycle.57,58 Both of these genes encode nuclear phosphoproteins that regulate cell cycle progression. When the RB protein (pRB) is in its hypophosphorylated form it inhibits entry of the cell into the S phase of the cell cycle by binding a transcription factor transcription factor E2 promoter‐binding‐protein (E2F) that stimulates mitosis‐promoting genes (Figure 1.7). When a cell is stimulated to divide, pRB is hyperphosphorylated by cyclins, causing it to release E2F, which enables cells to enter the S phase. Following the S phase, pRB is dephosphorylated and is, once again, able to bind E2F and inhibit entry of the cell into the S phase. In tumor cells, the ability of pRB to bind E2F is disrupted and the checkpoint is eliminated. For example, oncogenic DNA viruses (discussed later) can disrupt cell cycle control by synthesizing viral proteins that block the uptake of transcription factors by pRb protein. Figure 1.7 Tumor suppressor protein pRB. When pRB is hyperphosphorylated by cyclin‐dependent kinases it releases members of the transcription factor E2F family that then bind to DNA and stimulate progress from G1 into the S phase of the cell cycle. When pRb is hypophosphorylated it binds E2F and interacts with histone‐modifying proteins, histone deacetylase and histone methyltransferase, inhibiting progress through the cell cycle. When the ability of pRB to bind E2F is disrupted by mutations or viruses, the checkpoint is eliminated and cells may then proliferate in an uncontrolled fashion. The TP53 gene encodes a nuclear phosphoprotein that can regulate movement of the cell through the cell cycle. Although this phosphoprotein (p53) is not involved in regulation of the normal cell cycle, it plays an important role in cells that have sustained genetic damage or in conditions of hypoxia. In the absence of functional p53 these genetically damaged cells may undergo neoplastic transformation. Through mechanisms that are not well understood, p53 can detect when a cell sustains genetic damage by UV light, irradiation, or carcinogenic chemicals and then arrests the entry of the cell into the S phase from the G1 phase of the cell cycle to allow time for the repair of cellular DNA damage via growth arrest and DNA damage‐inducible protein (GADD45), which allows for DNA repair and cyclin‐dependent kinase inhibitor 1 (CDKN1 or p21) that inhibits phosphorylation of cell cycle‐related kinases. If the extent of DNA damage is too excessive, p53 can promote cellular apoptosis. Although normally a short‐lived protein, after genetic damage, p53 is modified in a way that causes it to have a significantly longer half‐life. P53 will then accumulate in the nucleus, leading to cell cycle arrest by activating transcription of genes that inhibit specific cyclin‐dependent kinases and prevent the phosphorylation of the RB protein. Other effects include expression of genes involved in DNA repair or apoptosis. Cells carrying mutated TP53 genes or cells infected with oncogenic DNA viruses that alter the function of p53 do not arrest before entering the S phase of the cell cycle and are less likely to undergo apoptosis (Figure 1.8). Affected cells can continue to replicate with damaged DNA, and those that do not develop lethal genetic changes are at risk for acquiring additional genetic damage, leading to neoplastic transformation. Figure 1.8 Tumor suppressor protein, p53. The tumor suppressor gene (TP53) encodes a protein, p53, which is crucial for repair or apoptosis of genetically damaged cells. Signaling is mediated through growth arrest and DNA damage‐inducible protein (GADD45) that allows for DNA repair and cyclin‐dependent kinase inhibitor 1 (CDKN1 or p21) that inhibits phosphorylation of cell cycle‐related kinases and arrests progression through the cell cycle. When genetic damage is too severe to be repaired p53 can initiate apoptosis via activation of the apoptosis‐stimulating gene BAX. Alternatively, activation of p53 in severely damaged cells can also trigger transcription of microRNAs (miRNA) that drive cell senescence. When TP53 is damaged by chemicals, radiation, viruses, or inherited defects, p53 production may be abrogated or a mutant p53 protein produced. Mutant p53 does not function normally and affected cells with damaged DNA do not arrest the cell cycle to enable DNA repair. Mutated cells are able to progress though the cell cycle giving rise to daughter cells with mutations and eventual tumor formation. Thus, when the gene TP53 is damaged or absent, tumor suppression is compromised. The canine DNA sequence for TP53 is 87% identical to the human sequence and has a similar intracellular role.59.60 Because mutations in TP53 occur in a high proportion (approximately 50%) of some types of human neoplasms, the frequency of TP53 mutations in animals has been examined. Mutations in TP53 of dogs have been detected most often in osteosarcomas, where they can be detected in approximately 40% of cases.61 In canine melanomas mutations have been identified in several tumor suppressor genes, including TP53.62 TP53 is also found to have altered expression or to be mutated infrequently in several types of canine and feline neoplasms, including canine mammary tumor,63 canine and feline squamous cell carcinoma,21,64 and canine mastocytoma.65 Cytogenetic analysis has demonstrated loss of several tumor suppressor genes in canine histiocytic sarcoma.66,67 Genes that control programmed cell death play a significant role in tumor development when they fail to function normally.68 B‐cell lymphomas serve as examples of the importance of the genes that control apoptosis. These tumors are characterized by an increased expression of the gene BCL2 (derived from B‐cell lymphoma 2), which blocks apoptosis. BCL2 is only one of a family of genes that participate in the regulation of apoptosis. The ability of oncoproteins such as BCL2 to block cell death pathways may enable cells that have sustained genetic damage to escape mechanisms that would stimulate normal cells to undergo programmed cell death. Alternatively, neoplastic lymphocytes that overexpress BCL2 can persist and slowly form lymphoid masses, unlike normal lymphoid cells that have a finite lifespan. Consequently, cells eluding apoptosis could multiply and are at risk to accumulate additional genetic damage that can heighten malignancy. Overexpression of BCL2 has been demonstrated in feline lymphoma, but was not associated with prognosis.69 Essentially unlimited replicative potential is a key feature in the formation of malignancies.48,70 While normal cells are capable of no more than 60–70 doublings before they become senescent and die, malignant cells must be free of these growth constraints in order to continually grow and expand. The principal mechanism by which cells replicate without entering senescence involves the enzyme telomerase. Telomerase is typically inactive in somatic cells but is active in stem cells, germ cells, and cancer cells. Activation of telomerase is recognized in about 85–95% of human cancers.70,71 Telomerase is a ribonucleoprotein complex that includes a reverse transcriptase, an RNA template, and additional proteins (Figure 1.9). This complex is responsible for adding back short sections of DNA of 50–200 nucleotides that are lost from the chromosomal telomeres (specialized nucleoprotein structures at the ends of chromosomes) during normal DNA replication cycles. Continued loss of the ends of chromosomes in normal cells will eventually trigger senescence and apoptosis by activating tumor suppressor genes encoding TP53 and pRb. Cells that lack normal tumor suppressor gene activity do not arrest at appropriate cell cycle checkpoints, leading to acquisition of various mutations. Telomerase activity has not been extensively studied in veterinary oncology. There is evidence from one canine study that nearly all lymph nodes (97%) with a histologically confirmed diagnosis of lymphoma (various subtypes) had detectable telomerase activity and activity was greater than that seen in normal lymph nodes.72 More work in this area is needed. Figure 1.9 Telomerase. Telomerase is an enzyme that enables cells to replicate in an unlimited fashion. Cells with repressed telomerase activity such as somatic cells eventually reach senescence after a finite number of mitoses and undergo cellular senescence, a permanent growth arrest state, or apoptosis. Telomerase adds back short sections of DNA that were lost from the chromosomal telomeres (repetitive nucleoprotein sequences at the ends of chromosomes) during normal DNA replication cycles. Cells with telomerase activity such as stem cells, germ cells and cancer cells can potentially proliferate indefinitely and are potentially immortal. Solid neoplasms depend on the blood vessels and supporting stroma that they recruit from adjacent tissue for their survival and growth.48,73 Without vascularization, growing tumor masses are limited to about a 1–2 mm diameter. In normal tissue and in neoplastic masses angiogenesis is regulated by competing pro‐ and anti‐angiogenic signaling. The transition to a pro‐angiogenic status occurs when anti‐angiogenic signaling is overwhelmed. Tumor cells secrete growth factors such as vascular endothelial growth factor A (VEGF‐A) and various types of fibroblastic growth factors (FGF) or stimulate other cells to release angiogenic factors that stimulate the vessels and supporting stroma in tumors (Figure 1.10). There are many cell types that participate in angiogenesis. In addition to the tumor cells and adjacent stromal elements, bone marrow–derived cells, primarily cells of the innate immune system, macrophages, neutrophils, and mast cells, and also myeloid precursors infiltrating at the margins of neoplastic lesions release angiogenic factors contributing to the ingrowth of new vessels.73 Angiogenesis was once thought to be significant primarily when robust tumor growth was occurring, yet is now known to begin early in the process of tumorigenesis and is evident in preneoplastic and benign lesions.74 The processes of angiogenesis and stroma formation are similar in tumors and wound healing, leading to the conceptual description of tumors as nonhealing wounds. There are some distinct differences in the structure and function of the vessels that are formed during each process.75 In tumors, the blood vessels are poorly differentiated and are not distributed uniformly through the tumor. Tumor blood vessels tend to be more tortuous and dilated than normal vessels, with gaps in the endothelium rendering them persistently permeable, unlike vessels in healing wounds that have a transient phase of permeability.76 Since most tumor cell entry into the bloodstream occurs between gaps in the endothelial cells it is likely that metastasis is facilitated by these abnormal vessels. Increased interstitial pressure due to the permeable vessels and the lack of lymphatics to carry away the leaked fluid lead to edema formation. This edema and the resultant interstitial fluid pressure tend to collapse the vessels within the tumor, thus obstructing local blood flow. The density of vascular supply to tumors is frequently minimally adequate and is deficient in arteriolar supply, in particular. As a result, irregular blood flow and perfusion cause localized areas of hypoxia and anoxia, leading to apoptosis or necrosis.
An Overview of Molecular Cancer Pathogenesis, Prognosis, and Diagnosis
Fundamentals of cancer biology
Genetic injury
Epigenetics
Tumor heterogeneity, tumor progression, and clonal evolution
The hallmarks of malignancy
Sustaining proliferative signaling: proto‐oncogenes and oncogenes
Classification of oncogenes
Alterations of gene structure (function)
Chromosomal translocation
Alterations of gene expression
MicroRNAs
Oncogenic viruses
Tumor proliferation
Evading growth suppressors
Resisting cell death
Enabling reproductive immortality
Inducing angiogenesis
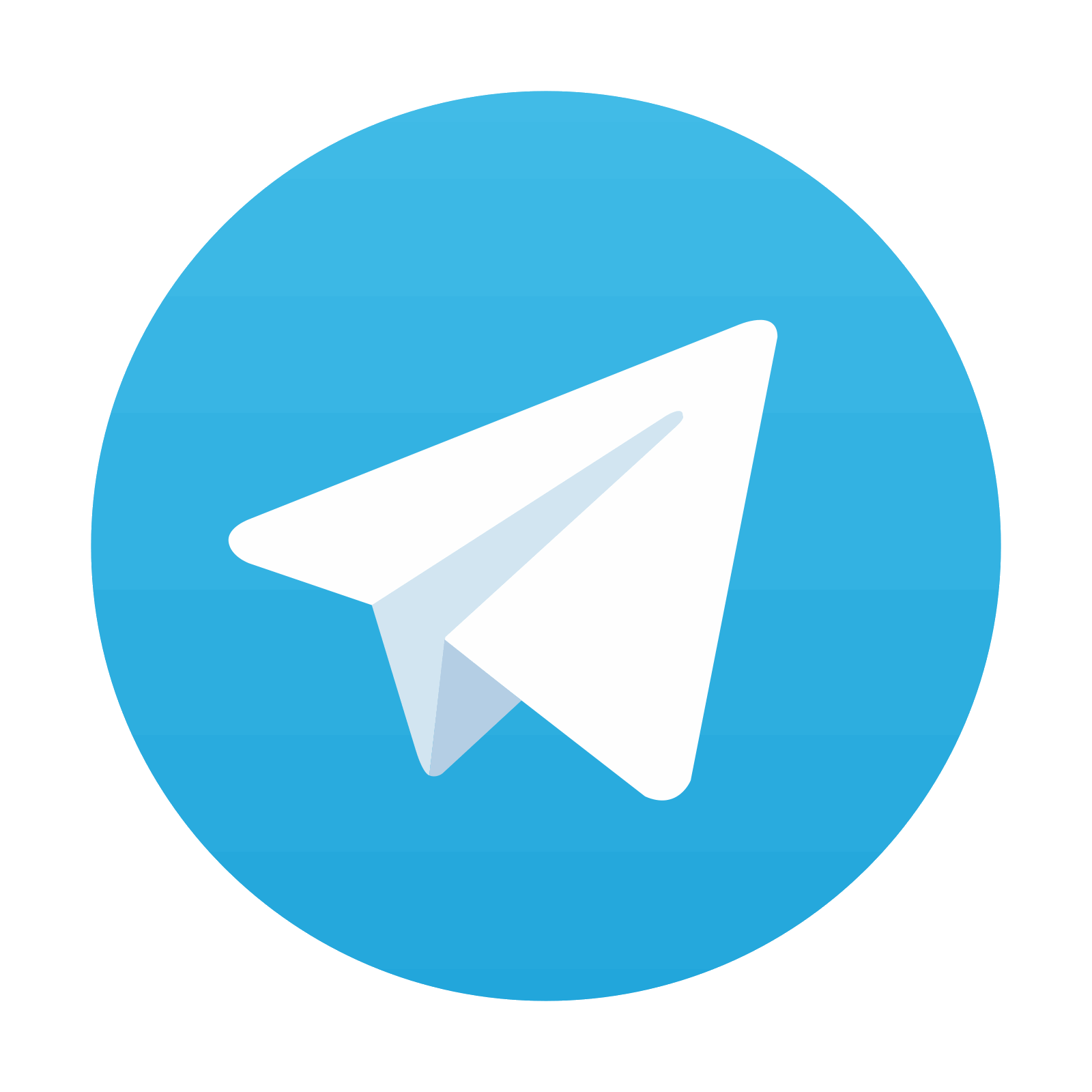
Stay updated, free articles. Join our Telegram channel
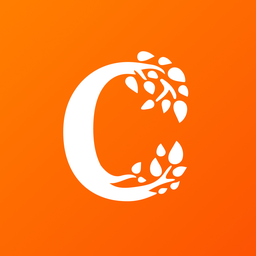
Full access? Get Clinical Tree
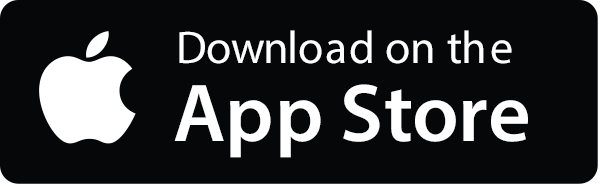
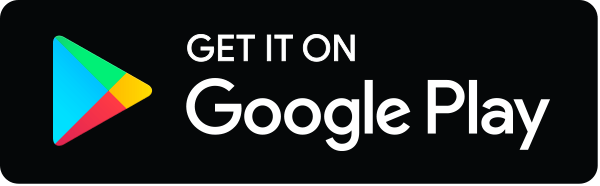