Introduction
Homeostasis requires maintenance of a relatively constant fluid volume and stable composition in all fluid compartments. The practice of anesthesia, even in American Society of Anesthesiologists (ASA) Category I patients undergoing routine procedures, may precipitate abnormalities in this balance. In patients presenting with critical illness, all fluid compartments may be significantly affected, and serious, potentially life-threatening derangements in acid–base balance may exist. These abnormalities will be further exacerbated by the administration of anesthetic agents if efforts to accurately diagnose underlying conditions, quantify fluid and acid–base disorders, and institute appropriate management strategies are not made.
The interplay between acid–base balance and fluid therapy is complex and injudicious administration of parenteral fluids during the perianesthetic period can have a detrimental effect on a patient’s acid–base status. In this chapter, basic principles of acid–base balance and fluid therapy will be presented as well as clinical guidelines for diagnosing acid–base disorders and providing appropriate perianesthetic fluid therapy to canine and feline patients.
Regulation of acid–base balance
Animals produce large quantities of acid through their metabolic processes every day that must be either excreted or metabolized to maintain acid–base balance. Despite this substantial production, the hydrogen ion concentration in body fluids is maintained at an extremely low level (40 nEq/L). Because the hydrogen ion exists in such miniscule concentrations, even small variations will have significant effects. For example, a change in hydrogen ion concentration from 35 to 45 nEq/L is associated with a change in pH of only 0.1 units, but this represents a 22% increase in its concentration.
The importance of the hydrogen ion in maintenance of homeostasis is not a function of its concentration in a particular fluid compartment but rather its impact on ionization. This is most critical within the intracellular space. Virtually all small metabolic intermediate molecules, with very few exceptions, are ionized at physiological pH. This results in ion trapping, which minimizes diffusional loss and is crucial to the maintenance of cellular homeostasis. In addition, ionization of amino acid residues (especially histidine) on larger protein molecules (notably enzymes) determines the three-dimensional shape of the molecules and affects their binding characteristics and overall function.
Acid–base terminology
Hydrogen ion (H+) A single free proton released from a hydrogen atom; note that “bare“ protons do not actually exist in solution as they react with surrounding water molecules; however, this symbolic notation continues to be used out of convenience.
Acid (HA) A molecule containing hydrogen atoms that can release H+.
Base (A–) An ion or molecule that can accept H+.
pH The logarithmic scale conventionally used to express H+ concentration ([H+]) in solutions; pH is related to [H+] (expressed as equivalents per liter) as follows:
For example, normal [H+] is 40nEq/L; therefore:
Strong acid An acid that rapidly dissociates and releases large amounts of H+ in solution; for example, hydrochloric acid (HCl → H+ + Cl–).
Weak acid An acid that dissociates incompletely, releasing limited H+ in solution; for example, carbonic acid (H2CO3 → H+ + HCO3–).
Strong base A base that reacts rapidly with H + removing it from solution; for example, hydroxide (OH– + H+ → H2O).
Weak base A base that binds weakly with H+ in solution; for example, bicarbonate (HCO3–+ H+ → H2CO3).
Acid dissociation constant (Ka) The equilibrium constant for dissociation reactions that provides a quantitative measure of the strength of an acid in solution
pKa The logarithmic scale conventionally used to express Ka values where pKa = –logKa; in general, weak acids have large pKa values while strong acids have small pKa values.
Henderson–Hasselbach equation An equation derived from Ka, which describes the amount of H+ available to react with bases:
Respiratory or volatile acid Acid that is excreted by the lungs, usually refers specifically to carbon dioxide (CO2); however, CO2 is not technically an acid (according to the Brønsted definition) because it lacks H+ and cannot be a proton donor, thus, CO2 is thought of as having the potential to create an equivalent amount of carbonic acid (H2CO3).
Metabolic or fixed acid All acids produced by the body that are not excreted by the lungs (i.e., any acid other than CO2/H2CO3), often referred to by their anions (e.g., lactate, phosphate, sulfate, acetoacetate, or β-hydroxybutyrate) instead of their acids (i.e., lactic acid, phosphoric acid, sulfuric acid, acetoacetic acid, and β-hydroxybutyric acid, respectively), which may be a source of confusion.
Acidosis An abnormal process or condition that would lower arterial pH if there were no secondary changes in response to the primary etiologic factor.
Alkalosis An abnormal process or condition that would raise arterial pH if there were no secondary changes in response to the primary etiologic factor.
Acidemia Low blood pH; the result of acidosis.
Alkalemia High blood pH; the result of alkalosis.
Systems responsible for maintaining hydrogen ion concentration
Regulation of [H+] in body fluids to prevent acidemia or alkalemia involves three primary mechanisms: (1) the chemical acid–base buffer systems in body fluids, (2) the respiratory center, and (3) the kidneys. The chemical buffer system responds to changes in [H+] within a fraction of a second and has a large capacity to bind H+ until other systems can respond and definitively re-establish acid–base balance. The second line of defense is the respiratory system, which acts within minutes to eliminate volatile acid (i.e., CO2) from the body via hyperventilation. The third line of defense is the kidneys and they are ultimately responsible for excretion of fixed acids and for initiating compensatory changes in plasma HCO3– concentration in the presence of respiratory acid–base disorders.
Chemical buffering to regulate acid–base balance
A buffer is defined as any substance that can reversibly bind H+ and a buffer solution consists of a weak acid and its conjugate base. A generic buffer reaction is represented as follows:
Free H+combines with the anionic base (A–) to form a weak acid (HA), which can either remain in this state or dissociate back to A– and H+ depending on the [H+]. A particular buffer is most effective when the local pH is within 1 pH unit of its pKa (Table 7.1).
Chemical buffer systems are located throughout the body in a range of fluids and tissues as shown in Table 7.1. The three most important chemical buffers are the bicarbonate, phosphate, and protein buffering systems.
Bicarbonate buffer system
The bicarbonate system is responsible for approximately 80% of extracellular fluid (ECF) buffering, and contributes between 10% and 15% to total chemical buffering capacity. The system consists of a water solution containing H2CO3 (a weak acid) and its conjugate base, HCO3−(usually as the sodium salt NaHCO3). The entire system may be represented as follows:
Table 7.1. The major body buffer systems

TBW, total body water.
a T he imidazole ring of histidine has a p Ka of 6.4–7.0 while the α-amino group has a pKa of 7.4–7.9.
The formation of H2CO3 from CO2 and H2O is catalyzed by the enzyme carbonic anhydrase, which is abundantly located in alveolar and renal tubular epithelial cells and in red blood cells. The H2CO3 then ionizes weakly to form very small amounts of H+ and HCO3–. The second component of the system, NaHCO3, ionizes almost completely, forming large amounts of HCO3 − and Na+.
Based on the low pKa value of 6.1 and the limited concentrations of the two principle elements of the system (H2CO3 and HCO3–), it would be reasonable to assume that the bicarbonate system lacks significant buffering power. However, because the system is “open at both ends“ (i.e., both pCO2 and [HCO3–] can be adjusted via respiratory and renal mechanisms, respectively), the effectiveness of the bicarbonate buffer system is greatly increased.
Phosphate buffer system
The phosphate buffer system is not important in the ECF but does play a major role in buffering renal tubular fluid and intracellular fluid (ICF). The main components of the system are H3PO4 (phosphoric acid, a triprotic weak acid) and its conjugate bases, H2PO4–, HPO42– and PO43– (dihydrogen phosphate, hydrogen phosphate, and phosphate, respectively, usually in the form of sodium salts). At physiological pH values, the prevailing two species are H2PO4– and HPO42–.
While the pKa for the phosphate buffer system is 6.8, making it a potentially significant buffer in the ECF, its concentration here is only a fraction of the concentration of the bicarbonate buffer, so its contribution to ECF buffering is negligible.
In contrast, this system plays a key role in urine because phosphate is greatly concentrated in renal tubules and tubular fluid has a lower pH than ECF, making it an ideal fluid compartment for this buffer. The phosphate system is also important in the ICF for similar reasons (i.e., high concentrations are available and ICF pH is very close to 6.8).
Protein buffer system
Proteins constitute the most plentiful buffers in body fluids. While plasma proteins, notably albumin, make a modest contribution to ECF buffering, it is the contribution of intracellular proteins, which is by far the most important. Approximately 60–70% of the body‘s total chemical buffering capacity occurs in the ICF and most of this is attributed to proteins. This is a function of the high concentration of protein found in cells and the fact that most protein buffers have pKa values fairly close to that of ICF. The most important protein-dissociable group is the imidazole ring of histidine (pKa 6.4 to 7.0), while α-amino groups (pKa 7.4–7.9) also play an important secondary role.
Hemoglobin is a key intracellular protein buffer. Because it is confined to red blood cells, it is also often classified as a blood buffer along with plasma proteins. Hemoglobin is responsible for more than 80% of the nonbicarbonate buffering capacity of whole blood while albumin makes up most of the other 20%. This is because hemoglobin is present in about twice the concentration and contains about three times the number of histidine residues per molecule compared to albumin.
Deoxyhemoglobin is a more effective buffer than oxyhemoglobin and this accounts for about 30% of the Haldane effect (the greater ability of deoxyhemoglobin to form carbamino compounds accounts for the other 70%). This means that oxygen unloading actually improves buffering capacity at exactly the location where additional H+ is being produced by dissociation of H2CO3 to facilitate CO2 transport as HCO3–.
Other factors impacting intracellular buffering
While the previous discussion has referred to the ECF and ICF as two totally distinct compartments, it is important to recognize that changes in [H+] are effectively communicated between them. Transfer of key elements across cell membranes has a significant impact on buffering and is facilitated by three major processes.
First, due to its high lipid solubility, CO2 crosses cell membranes easily where it dissolves in H2O to form H2CO3 –, which then dissociates to H+ and HCO3–. Due to the bicarbonate system’s ineffectiveness at buffering CO2 (i.e., volatile acid) in the ECF, almost all buffering of respiratory acidosis and alkalosis occurs intracellularly.
Second, cell membranes contain various proton–cation antiporters, which facilitate entry of H+ into cells in exchange for other cations, such as Na+ and K+, to maintain electroneutrality. The delivered H+ are then buffered by the phosphate and protein buffer systems. More than half of buffering for metabolic acidosis and about one-third of buffering for metabolic alkalosis occurs intracellularly.
Finally, the presence of the HCO3–/Cl – antiporter (or chloride pump) facilitates the movement of HCO3 – produced from the dissociation of H2CO3 out of the cell in exchange for Cl–. This is referred to as the chloride shift.
The isohydric principle
Chemical buffer systems do not operate independently but work together in equilibrium with each other. Because there is only one [H+] in a given compartment, anything that changes the balance of one buffer system will affect the balance of all the others. This is known as the isohydric principle. Clinically, this means that measuring the concentrations of any one acid–base pair will provide a picture of overall acid–base balance in the body. Conventionally, the components of the bicarbonate system (i.e., H2CO3 measured as pCO2, and HCO3–) are measured because they are accessible and easily determined. Blood gas machines measure pH and pCO2 directly and the [HCO3–] is then calculated using the Henderson–Hasselbalch equation.
Respiratory regulation of acid–base balance
The second line of defense against acid–base abnormalities involves the respiratory system, which is able to excrete volatile acid (CO2) and control [H+] by initiating changes in ventilation. Ventilatory changes occur rapidly and have profound effects on [H+]. Changes in alveolar ventilation are inversely proportional to changes in arterial pCO2 (PaCO2) and directly proportional to total body CO2 production. Changes in PaCO2, in turn, are inversely proportional to pH according to the Henderson–Hasselbach equation. Therefore, a decrease in alveolar ventilation will cause an increase in PaCO2 and a decrease in pH, while an increase in alveolar ventilation will cause a decrease in PaCO2 and an increase in pH.
Not only does alveolar ventilation impact pH, but the reverse also occurs. A decrease in pH will stimulate ventilatory drive while an increase in pH will inhibit it. Thus, the respiratory system operates as a typical negative feedback loop to maintain PaCO2 and therefore [H+] within a narrow range. Peripheral and central chemoreceptors sense changes in PaCO2, the respiratory center of the medulla integrates this information, and the muscles of respiration respond accordingly. This type of acid–base regulation is referred to as physiological buffering because it is an open system, as opposed to chemical buffering, which is a closed system. In general, the overall buffering capacity of the respiratory system is one to two times greater than the buffering power of all ECF chemical buffers combined.
Renal regulation of acid–base balance
While the lungs are responsible for elimination of volatile acid (CO2), the kidneys serve as the primary means by which fixed, metabolic acids are eliminated from the body. Despite the fact that the amount of fixed acid produced daily is far, far smaller than the amount of CO2 produced, the renal system is critical as there is no other mechanism for excretion of these acids. Quantitatively, even more important than excretion of fixed acids is the kidneys’ ability to reabsorb bicarbonate, thereby conserving the primary buffer system of the ECF. The kidneys accomplish these two goals through three fundamental processes: (1) active secretion of H+ into the tubular lumen, (2) indirect reabsorption of filtered HCO3– into tubular cells and ultimately the blood, and (3) production of new HCO3– in tubular cells involving the phosphate and ammonia buffer systems.
The first two mechanisms are interrelated in that the process of active H+ secretion into the renal tubular lumen ultimately results in reabsorption of HCO3–. The cellular mechanisms of H+ secretion depend on the location within the kidney. In the proximal tubules, the thick ascending loop of Henle and the early distal tubule, H+ is secreted via secondary active transport. In the intercalated cells, H+ is secreted by primary active transport.
In both cases secreted H+ combines with filtered HCO3–, forming H2CO3, which dissociates into CO2 and H2O. The CO2 diffuses easily into the tubular cell where it recombines with H2O under the influence of carbonic anhydrase to generate a new H2CO3 molecule, which in turn dissociates into HCO3– and H+. The HCO3– is then transported across the basolateral membrane by either Na+–HCO3– cotransport or Cl––HCO3– exchange. Under normal circumstances, each time an H+ is formed in a tubular cell an HCO3– is also formed and ultimately released back into the blood. Therefore, it can be said that HCO3– and H+ “titrate“ each other in the tubules.
In acidosis, there is excess H+ relative to HCO3– but only a small part of this can be excreted in the ionic form, as urine pH has a lower limit of approximately 4.5. Consequently, excretion of large amounts of urine H+ is accomplished by combining it with phosphate and ammonia buffers in the tubular lumen. This buffering actually results in the formation of “new“ (i.e., not previously filtered) HCO3–, which ultimately enters the blood. The ammonia system is quantitatively more important than the urinary phosphate system. In the proximal tubular cells, glutamine is metabolized to form ammonium and HCO3–. The ammonium is secreted into the tubular lumen while the new HCO3– is transported back across the basolateral membrane. In the collecting tubules, H+ is secreted into the tubular lumen as described previously, but now it combines with ammonia, which is readily able to diffuse across the luminal membrane. The result is ammonium, which is much less diffusable and becomes trapped in the urine, thereby providing a means of H+ excretion. For each ammonium excreted, a new HCO3– is formed and added back to the blood.
Clinically, it is possible to quantify net renal acid excretion by determining HCO3– excretion, the amount of new HCO3– added to the blood (which is calculated by measuring urinary ammonium concentration), and urinary titratable acid. Titratable acid refers to the rest of the non-HCO3–, nonammonium buffer excreted in the urine (i.e., mainly phosphate).
As secretion of H+ into the tubular lumen is a key step in all aspects of renal acid–base balance, it is not surprising that this process is tightly regulated. Key factors that may increase or decrease H+ secretion include changes in the following: pCO2, [H+], ECF fluid volume, angiotensin II levels, aldosterone levels, and potassium levels.
Temperature effects on acid–base balance
Evaluations of acid–base balance in a clinical setting typically involve measurement of ECF components simply because blood is an easily accessible fluid. However, it has been suggested that the intracellular environment is, in fact, the key compartment due to the phenomenon of ion trapping. There are two competing theories that seek to explain the regulation of intracellular pH across variations in temperature, the alpha-stat hypothesis, and the pH-stat hypothesis. While an in-depth discussion of these theories is not possible here, a brief definition of each is provided as they are relevant to the clinical interpretation of blood gas values.
The alpha-stat hypothesis states that the degree of ionization (referred to as “alpha“) of the imidazole groups of intracellular proteins remains constant despite changes in temperature. In other words, the ideal intracellular pH is the pH of neutrality (i.e., the state where [H+] = [OH–]) and this pH will vary with changes in temperature. If the alpha-stat theory is correct, then blood gas values should not be corrected for the patient‘s body temperature.
The pH-stat theory states that the intracellular pH should be kept constant despite changes in temperature. If the pH-stat theory is correct, then temperature-corrected blood gas values should be used for interpretation. It should be noted that all blood gas analyzers are set to 37 °C and all measurements are performed at this temperature. When other patient temperatures are entered the computer in the machine uses various correction formulae to calculate the so-called corrected values.
While both approaches continue to be used in clinical practice, the reality is, with significant variations in patient temperature, we simply do not fully understand the complexity of effects on cellular metabolism, vascular function, and respiration. Therefore, until definitive evidence is produced, both corrected and uncorrected blood gas values are of questionable utility in patients with significant deviations from 37°C.
The traditional approach to acid–base analysis
Any approach to clinical acid–base analysis must accurately identify the presence of an abnormality, quantify its magnitude, and offer insight into the nature and underlying cause. Acid–base physiology and analysis has a long history with over a hundred years of research and new additions to the literature every day. Newer approaches tend to build on or are modifications of previous approaches, so it is not surprising that the evolution of acid–base theory has led to considerable confusion for students and clinicians alike.
The first method that evolved is now referred to as the traditional or bicarbonate-based approach. It emerged from the work of Henderson and Hasselbach in the early 1900s and focuses on pCO2 and HCO3– as key elements. This approach is responsible for much of the conventional acid–base terminology that is still commonly found in textbooks and continues to be used in many clinical situations. Consequently, a brief discussion of the traditional approach and related terminology will be included here.
Table 7.2. Causes of acid–base abnormalities in dogs and cats classified according to the traditional approach
Metabolic acidosis |
• Diarrhea |
• Renal tubular acidosis |
• Dilutional acidosis (rapid 0.9% NaCl administration) |
• Carbonic anhydrase inhibitors |
• Ammonium chloride administration |
• Cationic amino acid administration |
• Hypoadrenocorticism |
• Lactic acidosis |
• Uremic acidosis |
• Diabetic ketoacidosis |
• Hyperphosphatemia |
• Intoxication |
– ethylene glycol |
– salicylates |
– metaldehyde |
Metabolic alkalosis |
• Vomiting of stomach contents |
• Gastric suctioning |
• Thiazide or loop diuretics |
• Posthypercapneic alkalosis |
• Primary hyperaldosteronism |
• Hyperadrenocorticism |
• Oral administration of NaHCO3– |
• Oral administration of other organic anions |
• Refeeding syndrome |
• High dose penicillin derivative antibiotics |
• Severe potassium or magnesium deficiency |
Respiratory acidosis |
• Airway obstruction |
– For example, aspiration, mass, tracheal collapse |
• Central respiratory depression |
– For example, drug induced, neurological disease |
• Neuromuscular disease |
– For example, myasthenia gravis, tetanus |
• Increased CO2 production with impaired ventilation |
– For example, heat stroke, malignant hyperthermia, cardiac arrest |
• Restrictive extrapulmonary disorders |
– For example, diaphragmatic hernia, pleural space disease |
• Intrinsic pulmonary disease |
– For example, pneumonia, pulmonary thromboembolism |
• Ineffective mechanical ventilation |
• Marked obesity (Pickwickian syndrome) |
Respiratory alkalosis |
• Hypoxia |
– For example, severe hypotension, severe anemia, congestive heart failure |
• Pulmonary parenchymal disease |
– For example, pneumonia, pulmonary thromboembolism |
• Centrally mediated hyperventilation |
– For example, liver disease, hyperadrenocortism |
• Systemic inflammatory response syndrome |
• Exercise, excitement, pain, anxiety |
• Overzealous mechanical ventilation |
Simple acid–base disorders
A simple acid–base disorder is one where there is a single primary etiologic disturbance. According to the traditional approach, there are four simple acid–base disorders to consider: (1) respiratory acidosis, (2) metabolic acidosis, (3) respiratory alkalosis, and (4) metabolic alkalosis. Respiratory disorders are caused by abnormal processes that alter pCO2 levels, while metabolic disorders are caused by abnormal processes that alter [HCO3–]. A list of common causes of the four primary acid–base disorders in dogs and cats classified according to this approach can be found in Table 7.2.
Secondary or compensatory acid–base changes usually occur in response to most primary acid–base abnormalities in an effort to restore [H+] to normal. In simple acid base disorders, these compensatory responses are predictable and a stepwise analysis is conducted according to Figure 7.1. With primary metabolic abnormalities, respiratory compensation involves either hyper- or hypoventilation. In most cases, the respiratory response occurs rapidly and is complete within hours, assuming the metabolic disturbance remains stable. With primary respiratory abnormalities, the compensatory response is biphasic. Acutely, the metabolic response is limited to chemical buffering, which may have a very modest impact on [HCO3–]. With more chronic respiratory disorders, the kidneys respond and are able to excrete or reabsorb HCO3– as appropriate. This renal response is slow to be initiated and takes 2–5 days to reach its maximum potential.
The degree of expected compensation for acid–base disorders varies among species. In clinical canine patients, guidelines derived from healthy experimental dogs are commonly used and are presented in Table 7.3. There is limited data available for evaluating compensatory responses in cats but experimental evidence based on a small number of animals suggests cats do not appear to initiate a ventilatory response to metabolic acidosis. Consequently, extrapolation of canine values to feline patients may be misleading and cannot be recommended.
Table 7.3. Expected compensatory changes to primary acid–base disorders in dogs according to the traditional approach
Primary disorder | Expected compensation |
Metabolic acidosis | ↓ Pa CO2 of 0.7 mm Hg per 1 mEq/L ↓ HCO3– 3 |
Metabolic alkalosis | ↑ Pa CO2 of 0.7 mm Hg per 1 mEq/L ↑ HCO3– 3 |
Respiratory acidosis (acute) | ↑ HCO3– of 0.15 mEq/L per 1 mm Hg ↑ Pa CO2 2 |
Respiratory acidosis (chronic) | ↑ HCO3– of 0.35 mEq/L per 1 mm Hg ↑ Pa CO2 2 |
Respiratory alkalosis (acute) | ↓ HCO3− of 0.25 mEq/L per 1 mm Hg ↓ Pa CO2 2 |
Respiratory alkalosis (chronic) | ↓ HCO3– of 0.55 mEq/L per 1 mm Hg ↓ Pa CO2 2 |
Mixed acid–base disorders
A mixed acid–base disorder is present when there are two or more primary etiologic disturbances present simultaneously. Mixed disorders may be additive (such as respiratory and metabolic acidosis) or offsetting (such as respiratory alkalosis and metabolic acidosis) with regard to their effects on pH. A triple disorder is an example of a complex acid–base disorder where a respiratory disturbance further complicates concurrent metabolic acidosis and metabolic alkalosis. With the traditional approach, differentiating a mixed acid–base disorder from a simple disorder and its compensatory response is accomplished by evaluating the magnitude of the compensation as discussed above and shown in Table 7.3. If the compensatory response is not within the range expected for the primary disorder then a mixed disorder is diagnosed. Caution must be exercised when evaluating the appropriateness of metabolic compensation to a primary respiratory disorder as it will depend on the chronicity of the respiratory abnormality, which may be difficult to ascertain clinically.
In general, the possibility of a mixed acid–base disorder should be suspected if one or more of the following are observed: (1) the presence of a normal pH with abnormal PCO2 and/or [HCO3–], (2) a pH change in a direction opposite that predicted for the known primary disorder, and (3) pCO2 and [HCO3–] changing in opposite directions.
Mixed acid–base disorders are more difficult to decipher than simple disorders and a diagnosis must be made in the context of the patient’s clinical signs and other diagnostic information. In most cases, the clinical significance of the acid–base perturbations will be a function of the underlying cause(s), which should become the focus of treatment.
Limitations of the traditional approach
While intuitively appealing and relatively simple in nature, the traditional bicarbonate-based approach remains essentially descriptive. In the mid-1960s, a group of researchers expanded on this concept in an attempt to better quantify and predict the nature of various acid–base disturbances. This approach, using acid–base maps or nomograms, became known as the Boston approach and these nomograms are still found in many textbooks. Unfortunately, the Boston/traditional approach has significant limitations. It fails to take into account the influence of nonbicarbonate buffer systems (i.e., plasma proteins and phosphate) as well as the effect of serum electrolytes (notably Na+, K+. and Cl–). Also, it incorrectly assumes that both pCO2 and HCO3– are independent variables when in fact only pCO2 is independent.
Consequently, the traditional approach is unable to provide insight into complex mixed acid–base disorders and can actually be misleading in certain circumstances. Metabolic acidosis, in particular, poses significant problems for the traditional approach. This abnormality has been reported to be the most common acid–base disturbance in dogs and cats and accompanies such diverse conditions as sepsis, trauma, renal failure, and endocrine emergencies. In these settings, the traditional approach is not helpful. While it still retains a place in clinical acid–base analysis, its use should be reserved for patients with simple acid–base disorders and normal serum albumin and phosphate concentrations.
Alternative approaches to acid–base analysis
An increasing appreciation for the frequency and complexity of clinical acid–base disorders meant that better approaches for clinical assessment were sought. Highlights in the evolution of acid–base theory include the base excess approach, the anion gap approach, and the strong ion approach and its various modifications.
The base excess approach to acid–base analysis
The base excess approach (also referred to as the Copenhagen approach) was described by Sigaard-Andersen in the late 1950s as an attempt to better distinguish the relative contributions of the respiratory and metabolic components while still remaining faithful to the traditional concepts. The base deficit or excess (BDE) was defined as the amount of acid per unit volume that must be added to achieve a normal pH at a PaCO2 of 40 mm Hg using whole blood. This approach was later modified in the 1960s to use only serum base excess and the parameter became known as the standardized base excess (SBE]. Like the Boston group, the Copenhagen group generated data based on large numbers of human patients, leading to the development of SBE nomograms to facilitate clinical acid–base assessment. Most modern automated blood gas analyzers report an SBE value calculated from this human data. While a canine SBE nomogram has been developed using this approach, it is rarely used because commercial blood gas analyzers are programmed specifically for human blood. In a broader sense, this approach has also met with criticism because the SBE parameter represents the net effect of all metabolic acid–base abnormalities. In the case of coexisting metabolic acidosis and alkalosis, for example, SBE alone may fail to identify any acid–base abnormality as these two disturbances could apparently cancel each other out.
The anion gap approach to acid–base analysis
The anion gap approach was developed in 1975 by Emmit et al. and has proven to be a useful tool in the analysis of certain mixed acid–base disorders. The anion gap (AG) is calculated clinically as the difference between the major cations and anions that are commonly measured in plasma as shown below:
In reality, because electroneutrality must be maintained, there is no actual difference between the total number of cations and anions in plasma, so what the AG really represents is the difference between unmeasured cations and anions. Since changes in unmeasured cations of the magnitude necessary to impact AG do not occur clinically, AG is essentially a tool used to estimate the concentration of unmeasured anions.
Under normal circumstances, plasma proteins make up the majority of the unmeasured anions represented by the AG, and normal AG values are approximately 12–24mEq/L in dogs and 13–27mEq/L in cats. In metabolic acidosis, calculation of the AG may be useful in identifying the underlying cause, as different pathological states will impact the AG differently.
In the case of organic acidosis, fixed acids such as lactic acid or ketoacids are added to plasma where they rapidly dissociate into their anions (which are not measured as part of a typical biochemical profile) and H+ (which is buffered by HCO3–). The net effect is a decrease in measured anions (i.e., HCO3–) with no change in Cl–, leading to an increased AG. These types of acidosis are therefore classified as increased AG or normochloremic metabolic acidosis. In contrast, the loss of HCO3– that may accompany gastrointestinal fluid loss or renal tubular acidosis is associated with Cl– retention so the AG doesn’3 change. Consequently, these types of acidosis are classified as normal AG or hyperchloremic metabolic acidosis. Causes of metabolic acidosis classified according to the AG approach are shown in Table 7.4.
Table 7.4. Causes of metabolic acidosis in dogs and cats classified according to the AG approach
Metabolic acidosis |
Normal AG (hyperchloremia) |
• Diarrhea |
• Renal tubular acidosis |
• Dilutional acidosis (rapid 0.9% NaCl administration) |
• Carbonic anhydrase inhibitors |
• Ammonium chloride administration |
• Cationic amino acid administration |
• Hypoadrenocorticism |
Increased AG (normochloremia) |
• Lactic acidosis |
• Uremic acidosis |
• Diabetic ketoacidosis |
• Hyperphosphatemia |
• Intoxication |
– Ethylene glycol |
– Salicylates |
– Metaldehyde |
A significant factor that confounds interpretation of the AG is hypoalbuminemia. Since albumin is the major unmeasured anion in the normal state, the presence of hypoalbuminemia will decrease the AG and obscure the presence of anions, which would otherwise increase it. In other words, a patient with concurrent lactic acidosis and hypoalbuminemia may have a normal calculated AG and this would be clinically misleading. In dogs, the AG can be adjusted for changes in protein concentration by using the following formula:
While not as substantial as the effect of albumin, phosphate can also impact the AG. In dogs, the AG can be adjusted for both albumin and phosphate by using the following formula:
While the AG is a useful tool in certain situations it remains limited in its ability to elucidate complex mixed acid–base disorders. Attempts to optimize its usefulness have been explored in humans and have led to the concept of the delta ratio or the delta gap. Both of these parameters attempt to relate the change in AG to the change in [HCO3–], each from their respective reference values. Neither the delta ratio nor the delta gap has been studied in dogs or cats.
The strong ion approach to acid–base analysis
In 1983, Stewart published his now widely accepted model of acid–base physiology, which has been referred to by various names including the Stewart approach, the quantitative approach, the physicochemical approach, and the strong ion approach. According to Stewart there are three independent determinants of acid–base balance: (1) pCO2, (2) the difference between strong cations and strong anions (strong ion difference or SID), and (3) the total concentration of weak acids (ATOT). As is the case with the traditional approach, the pCO2 variable is the primary determinant of the respiratory component of plasma pH. The SID and ATOT variables provide independent measures of the metabolic component of plasma pH. Therefore, according to the strong ion approach, there are four possible primary metabolic acid–base disorders instead of only two with the traditional approach. Metabolic acidosis is caused by a decrease in SID or an increase in ATOT, while metabolic alkalosis is caused by an increase in SID or a decrease in ATOT. Please refer to Table 7.5 for common metabolic acid–base disorders classified according to the strong ion approach.
SID
Strong ions (also known as nonbuffer ions) are defined as any ion that is completely dissociated at physiological pH and include Na+, K+, Ca2+, Mg2+. and Cl+. as well as unmeasured ions such as lactate, β-hydroxybutyrate, acetoacetate, and sulfate. The SID is a term used to describe the difference between the sum of strong cations and the sum of strong anions. It is usually represented by the following equation (where [A–] refers to all unmeasured strong anions):
Table 7.5. Causes of metabolic acid–base abnormalities classified according to the strong ion approach
SID acidosis (↓ SID) |
Dilution acidosis (↓ Na+) |
• With hypervolemia |
– Severe liver disease |
– Congestive heart failure |
– Nephrotic syndrome |
• With normovolemia |
– Psychogenic polydipsia |
– Hypotonic fluid infusion |
• With hypovolemia |
– Vomiting |
– Diarrhea |
– Hypoadrenocorticism |
– Third space loss |
– Diuretic administration |
Hyperchloremic acidosis (↑ Cl–corr) |
• Loss of Na+ relative to Cl– |
– Diarrhea |
• Gain of Cl– relative to Na+ |
– Fluid therapy (0.9% NaCl, 7.2% NaCl, KCl-supplemented fluids |
• Cl– retention |
– Renal failure |
– Hypoadrenocorticism |
Organic acidosis (↑ unmeasured strong ions) |
• Uremic, keto- or lactic acidosis |
• Toxicities |
– Ethylene glycol |
– Salicylate |
SID alkalosis (↑ SID) |
Concentration alkalosis (↑ Na+) |
• Pure water loss |
– Water deprivation |
– Diabetes insipidus |
• Hypotonic fluid loss |
– Vomiting |
– Nonoliguric renal failure |
– Postobstructive diuresis |
Hypochloremic alkalosis (↓ Cl– corr) |
• Gain of Na+ relative to Cl– |
– 1sotonic or hypertonic |
NaHCO3– administration |
• Loss of Cl– relative to Na+ |
– Vomiting of stomach contents |
– Thiazide or loop diuretics |
ATOT acidosis (↑ ATOT ) |
Hyperalbuminemia |
• Water deprivation |
Hyperphosphatemia |
• Translocation |
– Tumor cell lysis |
– Tissue trauma/rhabdomyolysis |
• 1ncreased intake |
– Phosphate-containing enemas |
– 1ntravenous phosphate |
• Decreased loss |
– Renal failure |
– Urethral obstruction |
– Uroabdomen |
ATOT alkalosis (↓ATOT) |
Hypoalbuminemia |
• Decreased production |
– Chronic liver disease |
– Acute phase response to inflammation |
– Malnutrition/starvation |
• Extracorporeal loss |
– Protein-losing nephropathy |
– Protein-losing enteropathy |
• Sequestration |
– 1nflammatory effusions |
– Vasculitis |
Under physiologically normal conditions, the SID of plasma is approximately 40–44mEq/L and this difference is balanced by the negative charge on HCO3– and ATOT. Under pathological conditions, additional strong anions accumulate and [A–] becomes clinically significant, indicating organic acidosis. The SID may be calculated by measuring as many strong ions as possible and summing their charges. This original definition has now come to be known as the apparent SID (SIDapp), which distinguishes it from the effective SID (to be discussed later). With the capability to routinely measure lactate concentrations in the clinical setting, some recommend the addition of [lactate] to the SIDapp calculation as follows:
In fact, because Na+ and Cl– are quantitatively the most important strong ions, SIDapp is often further simplified in clinical situations as follows:
Concentration of weak acids (ATOT)
Stewart used the term ATOT to reflect the sum of all weak acids in both the dissociated and undissociated states (i.e., A– plus HA) according to the law of conservation of mass. The major contributors to ATOT are albumin, globulins, and inorganic phosphate, and these weak acids are also sometimes referred to as buffer ions. Changes in the concentrations of any of these, either directly or through changes to plasma free water content, will affect pH.
Simplifications of the strong ion approach
The strong ion approach provides a sound mechanistic framework to explain why plasma pH changes in any setting and successfully integrates electrolyte and acid–base physiology. Unfortunately, Stewart’s equations are mathematically complex and thus not easily applicable to the clinical setting. Consequently, a number of simplified modifications have been developed to facilitate clinical use of Stewart’s strong ion approach.
The effective SID modification
Some 10 years after Stewart published his strong ion theory, Figge et al. proposed a modification to Stewart’s approach, which attempts to quantify the effects of unmeasured strong anions using a parameter that he referred to as the strong ion gap (SIG). The term effective SID (SIDeff) was proposed to represent the contribution of weak acids. Therefore, unmeasured strong anions (i.e., the SIG) can be estimated by subtracting the SIDeff (calculated using formulas involving the weak acids HCO3–, albumin, and phosphate) from the SIDapp (calculated according to the equation presented previously). Despite its potential usefulness in humans, Figge’s formulas for calculation of SIDeff are complex and have not been evaluated in dogs and cats, so this approach is not currently feasible in veterinary medicine. The general concept of the SIG, however, has been revisited by others and will be discussed again below.
The base excess modification
In 1993, Fencl et al. published an additional modification of the Stewart approach that resurrected the concept of SBE and combined this with principles of the strong ion theory. The so-called Fencl–Stewart approach uses a set of equations to estimate the magnitude of effect of changes in SID and atot on the SBE value reported by blood gas analyzers. Equations for four distinct parameters have been proposed that can be calculated based on routine laboratory measurements: (1) the albumin effect or SBEAlb, (2) the phosphate effect or SBEPhos, (3) the free water effect or SBEFW (based on sodium), and (4) the chloride effect or SBECl. With the availability of lactate analyzers, a fifth parameter, the lactate effect or SBELact, can also be calculated. A sixth effect, the unmeasured strong ion effect or SBEXA, can then be calculated by subtracting the sum of the individual SBE effects from the reported SBE value or SBEreported (see Table 7.6). Some authors use different terminology and refer to the SBEXA as the base excess gap (BEG). Values of SBEXA less than –5 mEq/L are suggestive of an increase in unmeasured strong anions.
Table 7.6. Formulas for calculating contributions of key parameters according to the base excess modification of the strong ion approach
SBE effect (in mEq/L) | Formulas |
Effects relating to ATOT: | |
(1) SBEAlb | 3.7([AIbnormaI ref] – [Albmeasured]) in g/dL 0.37([AIbnormaI ref] – [Albmeasured]) in g/L]) |
(2) SBEphos | 1.8([PhosnormaI ref] – [Phosmeasured]) in mmol/L 0.58([PhosnormaI ref] – [Phosmeasured]) in mg/dL |
Effects relating to SID: | |
(3) sbefw | Dogs 0.25([Na+measured] – [Na+normal ref]) in mEq/L Cats 0.22([Na+measured] – [Na+normal ref]) in mEq/L |
(4) sbecl | [Cl–normal ref] – [Cl–corr] in mEq/L, where Cl– corr = [Cl– measured] x ([Na+normal ref]/[Na+measured]) |
(5) SBELact | –1[Lactmeasured] in mmol/L |
(6) sbexa (also known as BEG) | SBEreported – (SBEAlb + SBEPhos + sbefw + sbeci + SBELact) |
See text for additional definitions.
Albnormal ref, midpoint of the albumin reference range; Albmeasured, patient’ s albumin concentration; Phosnormal ref, midpoint of the phosphorous reference range; Phosmeasured, patient’s phosphorous concentration; Na+normal ref, midpoint of the sodium reference range; Na+measured, patient’ s sodium concentration; Cl–normal ref, midpoint of chloride reference range; Cl–corr, corrected chloride; Cl–measured, patient’s chloride concentration; Lactmeasured, patient’s lactate concentration.
Table 7.7. Summary of metabolic acid–base influences identified by the base excess modification of the strong ion approach
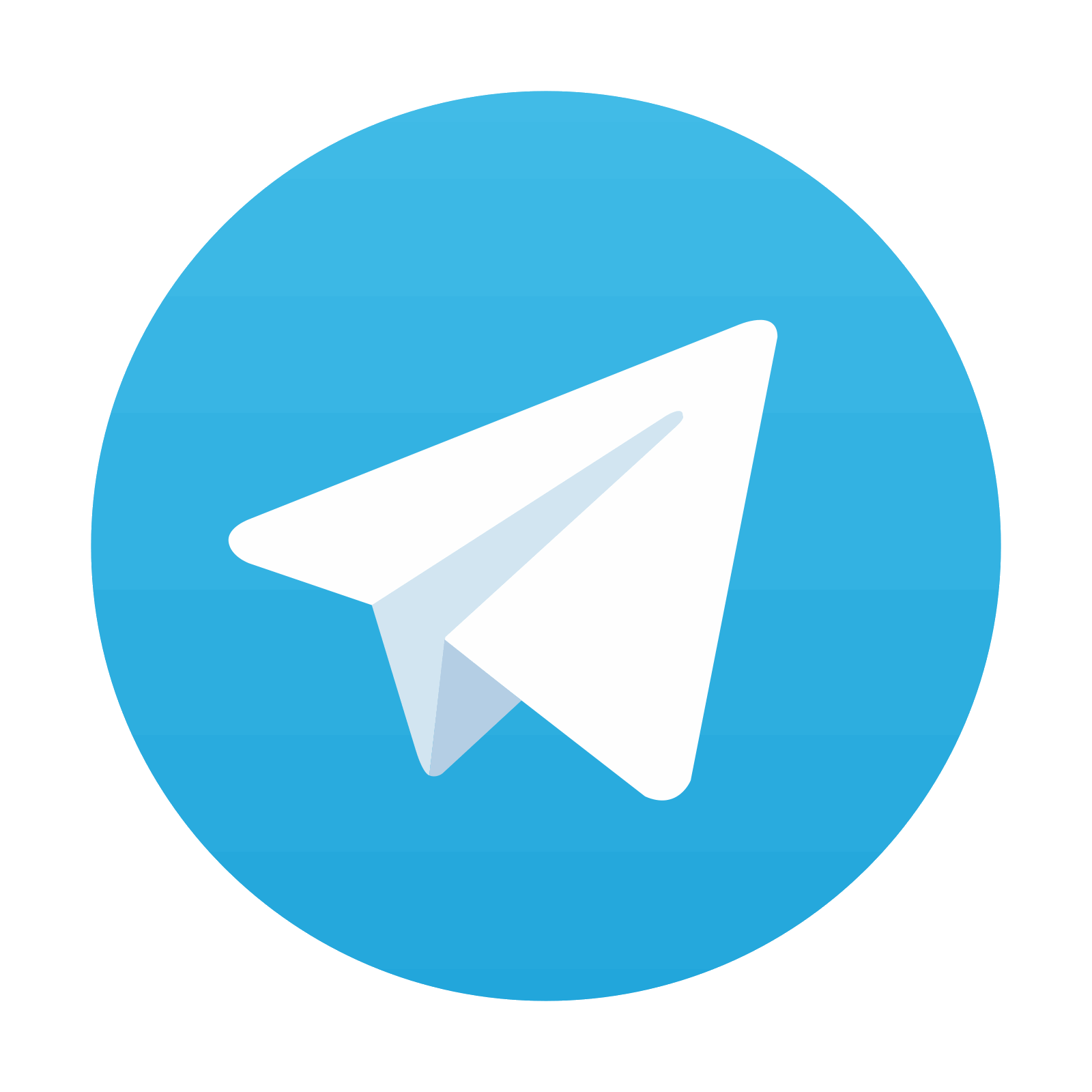
Stay updated, free articles. Join our Telegram channel
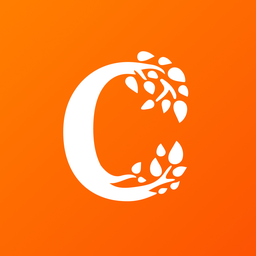
Full access? Get Clinical Tree
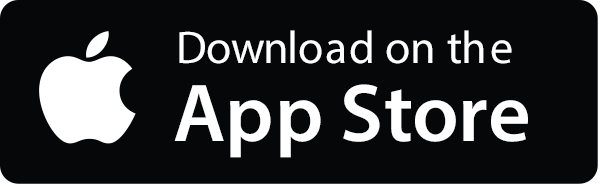
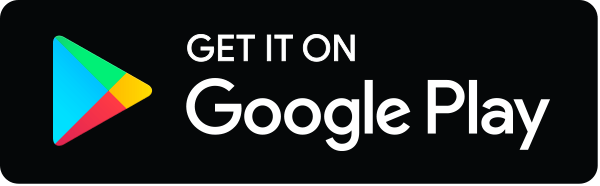