Chapter 67 Charlotte E. Farin, Callie V. Barnwell and William T. Farmer Department of Animal Science, North Carolina State University, Raleigh, North Carolina, USA Development of nonsurgical embryo transfer techniques1–3 led to the establishment of the bovine embryo transfer industry during the 1970s and 1980s. In nonsurgical embryo transfer, valuable female cattle are superovulated by treatment with follicle-stimulating hormone (FSH)-containing preparations, bred by artificial insemination (AI), and then subjected to nonsurgical uterine lavage, usually 7 days after insemination, to recover embryos at the morula and blastocyst stages of development. On average, five to seven embryos are recovered from a donor cow and individual embryos are then nonsurgically transferred to recipient females, who then carry the pregnancy to term. In 1997 approximately 360 000 bovine embryos produced in vivo, primarily from superovulated donor cows, were being transferred annually. By 2001, this number had risen to 452 546,4,5 and in the decade between 2001 and 2011 the number of commercial in vivo produced bovine embryo transfers roughly stabilized, averaging approximately 550 000 transfers annually worldwide.5,6 With the development of systems for in vitro, or laboratory-based, production of bovine embryos, hundreds of thousands of calves have resulted from the commercial transfer of in vitro produced (IVP) embryos. From 2001 through 2011, the number of commercial IVP bovine embryos transferred annually rose more than 10-fold, from a total of 30 260 transfers in 2001 to 373 869 in 2011 (Figure 67.1).5,6 Thus, in vitro embryo production and transfer now represents the actively growing segment of the commercial bovine embryo transfer industry. Advantages of using in vitro embryo production systems include the ability to produce embryos from valuable female cattle whose oviducts or uterus may have been damaged due to disease or dystocia as well as the ability to fertilize oocytes from a single collection of a donor female to different sires. In addition, the use of transferred IVP embryos is an effective method to improve pregnancy rate in dairy cows exposed to summer heat stress compared with that following AI.7–9 Figure 67.1 Number of commercial transfers of in vivo-produced or in vitro-produced bovine embryos worldwide between 2001 and 2011. In vitro production of bovine embryos is also a key technology supporting the application of more advanced embryo technologies such as gene transfer and somatic cell nuclear transfer (SCNT). From a commercial perspective, cloned cattle have been developed as bioreactors for producing biopharmaceuticals, critical human blood components, and modified milk components.10–14 In addition, genetic modification via nuclear transfer has been used to study the function and importance of individual genes and their involvement in diseases, developmental origins, and other physiological processes in both cattle and swine.15–19 SCNT has also been suggested as a potential technology that could be applied to rescue endangered or extinct species.20,21 Manipulation of bovine embryos became a reality with the development of techniques for embryo recovery and transfer. As noted above, the development of the commercial embryo transfer industry in cattle soon followed the introduction of nonsurgical embryo transfer techniques.1–3 Bovine embryos retrieved from donor dams could then be handled in the laboratory (in vitro) prior to their transfer to recipient females. Initially, embryos spent little time in the laboratory between transfers but as embryo culture medium was developed, embryos could be successfully maintained in the laboratory for a number of hours or even in overnight culture prior to transfer. In commercial practice, however, bovine embryos were rarely maintained outside the animal for longer than few hours prior to transfer. Assessment of development following transfer and calving supported the conclusion that normal development of offspring was associated with production by embryo transfer.22 Coincident with the commercialization of embryo transfer techniques in cattle was the advancement of manipulation-intense methods to study development of mammalian embryos in species such as mice23,24 and sheep.25,26 The intersection of investigations in these areas quickly led to the application of in vitro manipulation of bovine embryos in an attempt to improve efficiency of commercial production, initially through the application of embryo splitting and later through embryo cloning or nuclear transfer (NT). For splitting, embryos were recovered nonsurgically, typically at the morula to early blastocyst stage of development, bisected to produce identical twins and transferred within hours into suitable recipient females using nonsurgical methods.27–29 Splitting and other biopsy techniques were also applied for determination of embryo sex with one resulting demi-embryo transferred and the other portion used for sexing.30 As calves from these procedures continued to be produced, there were no reports of developmental abnormalities of offspring derived from the use of these methods. In contrast to reports for bisected bovine embryos, development of cattle embryos produced by NT using embryonic blastomeres as a source of donor nuclei26 resulted in reports of the production of oversized offspring and evidence of abnormal fetal and placental development.31–33 Coincident with the development of manipulation-intensive technologies such as embryo bisection and NT, techniques to successfully fertilize bovine oocytes in vitro 34 or successfully in vitro mature, in vitro fertilize, and in vitro culture bovine embryos up to the 8- to 16-cell stage of development were also established.35,36 However, few IVP bovine embryos were transferred because they could not be cultured beyond the 8- to 16-cell stage of development in vitro.37,38 Because nonsurgical embryo transfer had by then become the industry standard, only embryos at the morula (32–64 cells) or blastocyst (100–128+ cells) stages of development were considered suitable candidates for transfer. In the late 1980s, the use of oviductal cell coculture as a method to successfully overcome the developmental arrest of cultured bovine embryos at the 8- to 16-cell stage was established.39 As the decade of the 1990s progressed, blastocyst-stage bovine embryos could be routinely produced from bovine oocytes entirely matured in vitro, fertilized in vitro, and cultured in vitro.40–42 An early report of calf development following the transfer of IVP bovine embryos,38 although anecdotal, initially suggested a pattern of pregnancy loss during the second and third trimesters associated with the transfer of IVP bovine embryos. Similarly, a report of the development of sheep zygotes produced in vivo and recovered surgically from the oviduct that were then cultured in vitro for 5 days43 demonstrated that prolonged exposure of embryos to in vitro conditions prior to embryo transfer resulted in extended gestation lengths, increased lamb birthweights, and greater mortality compared with noncultured controls. In 1995, three papers were published44–46 documenting the occurrence of oversized fetuses or calves that resulted from the transfer of IVP bovine embryos. In addition, increased perinatal mortality was associated with fetuses and calves resulting from the transfer of embryos produced in vitro.44,45 In each of these cases, in vitro oocyte maturation was used in conjunction with in vitro fertilization and in vitro culture (IVC) for production of the embryos, fetuses and calves studied. This confounding made impossible the assignment of the effects of individual components of the in vitro process on the production of oversized fetuses and calves. Thus for cattle it was not possible to clearly determine if only IVC was responsible for production of oversized individuals as suggested from the data in sheep47 or whether other components of the in vitro production process also contributed. Collectively, however, these observations were the first to suggest that manipulation of the environment during bovine in vitro oocyte maturation, fertilization, and preimplantation culture contributed to alterations in subsequent gestational and perinatal development. Moreover, the developmental alterations were largely reminiscent of those observed in some of the offspring that resulted from blastomere cloning (NT)31–33 and, later, SCNT15,48,49 in cattle. As noted above, transfer of IVP, NT or SCNT manipulated bovine embryos results in a proportion of conceptuses, fetuses or offspring that exhibit developmental abnormalities or abnormalities in their associated placental tissues.32,44,45,50,51 Because the occurrence of an overgrowth phenotype of the fetus or offspring was the most striking observation initially identified as resulting from the transfer of either IVP or SCNT embryos, the phenomenon initially became known as “large offspring syndrome.”31–33,44,47,50 However, as more investigators began studying the phenomenon, it became clear that not all pregnancies from IVP and SCNT embryos resulted in phenotypes that exhibited excessive fetal overgrowth.52–58 Therefore, the term “abnormal offspring syndrome” (AOS) was used to more accurately describe the range of characteristics found associated with this syndrome.59 Designation of this syndrome as AOS is useful when discussing developmental anomalies associated with in vitro manipulation of embryos in other mammalian species. In swine, for example, an overgrowth phenotype was not associated with SCNT.60,61 However, cloned pigs can exhibit other developmental aberrations that would still be appropriately described as AOS.60 Because the etiology of AOS-like characteristics is associated with epigenetic alterations to the genome resulting from in vitro embryo manipulations, recognition that AOS occurs in a variety of mammalian species rather than only in cattle and sheep will assist in further defining all aspects of this syndrome. Characteristics associated with AOS in cattle have been grouped into four types59 (Figure 67.2). In type I AOS, abnormalities are exhibited in pregnancy establishment. In cattle, this is during the period between days 0 and 42 of gestation when organogenesis is occurring. In type II AOS, abnormalities occur that are associated with failure to maintain the fetoplacental unit resulting in abortion during the period following organogenesis until term, namely between days 42 and 280 of bovine gestation. In type III AOS, a range of abnormalities can occur in the fetoplacental unit that are either less severe or that permit compensation by the fetus and placenta, thus allowing the pregnancy to go to term; however, survival of the fetus during parturition or during the neonatal period does not occur, resulting in neonatal mortality. These compromised nonsurviving offspring exhibit altered clinical, hematological, or biochemical parameters. In type IV AOS, a range of abnormalities can occur in fetoplacental development that are either less severe or permit compensation by the fetus and placenta. In this case, the pregnancy succeeds in going to term and the neonatal calf survives; however, the offspring may still exhibit differences in anatomy, physiology, or metabolism compared with animals from nonmanipulated embryos. Figure 67.2 Classification of the subtypes of abnormal offspring syndrome (AOS). Production of embryos in vitro is associated with a greater incidence of early and mid-gestational loss.45,59 These cases represent type II AOS, with pregnancy losses typically occurring between days 40 and 90 of gestation.45 Similarly, the majority of SCNT pregnancies are lost between days 40 and 90 of gestation15,62 and would also be classified as type II AOS. In this case, losses are typically associated with improper development of the placenta.62 When SCNT pregnancies do survive, 65–70% of neonates exhibit some form of AOS and their condition would be classed as either type III or IV AOS. In some instances, intensive care is required to support survival of the neonate, without which the neonate typically does not survive (type III AOS), whereas in others little or no assistance is needed (type IV AOS).57 In cattle, the expression of anomalies associated with AOS can range from changes in gene expression in preimplantation-stage embryos63 to moderate alterations with little apparent phenotypic compromise57 to severe alteration in phenotype accompanied by abortion or neonatal death59,62,64 (Figure 67.3). Expression of AOS is influenced by culture conditions,58,65,66 species,61 extent of embryo manipulation,15,67 and even levels of maternal nutrients.68 At any specific stage of development, every embryo, fetus or calf does not exhibit a uniform set of AOS characteristics. Therefore, we have sought to compile observations reported as altered characteristics associated with the manipulation of bovine embryos in vitro at various stages of development. In each case, however, a direct correspondence between the type of manipulation, altered characteristic, and occurrence of AOS has not always been established. Figure 67.3 Identification of abnormalities commonly associated with abnormal offspring syndrome (AOS). In individual cases, each abnormality listed may or may not be present. There is a limited number of reports which suggest that alterations in the in vitro environment of spermatozoa prior to fertilization can be associated with abnormalities in subsequent embryo development.69,70 For example, exposure of bull sperm to polyvinylpyrrolidone (PVP), an agent commonly included in handling medium for intracytoplasmic sperm injection,70 increased the incidence of acrosome-reacted sperm following 30 or 60 min of PVP exposure and decreased the proportion of oocytes fertilized by PVP-exposed sperm following coincubation with in vitro matured oocytes.69 However, it is unclear whether exposure of sperm to PVP prior to fertilization had any effect on the subsequent development of the resulting zygotes in terms of morphology, time of cleavage, time of blastocoel formation, or inner cell mass and trophoblast cell number. Oocytes at the metaphase II stage of meiosis are ovulated from preovulatory follicles that, in cattle, typically range from 18 to 22 mm in diameter. In contrast to litter-bearing species such as swine, it is technically difficult to obtain large numbers of in vivo matured bovine oocytes for laboratory manipulations. Therefore, bovine oocytes used for both IVP and SCNT procedures are matured in vitro. Bovine oocytes used for in vitro production systems are derived from follicles usually 2–10 mm in diameter and are recovered at the dictyate stage within prophase I of meiosis. These dictyate oocytes must be subjected to in vitro maturation (IVM) for 20–24 hours during which they reach the metaphase II stage of meiosis and become ready for fertilization. Whereas 50–80% of in vivo matured oocytes develop to the blastocyst stage following fertilization, only 15–40% of in vitro matured oocytes reach the blastocyst stage in standard in vitro production systems.71–75 Furthermore, blastocysts from oocytes that were matured in vitro result in fewer numbers of cells per embryo75 and within the inner cell mass76–78 than blastocysts from oocytes matured in vivo. Similarly, development of transgenic blastocysts was greater from oocytes that were matured in vivo compared with oocytes that were matured in vitro.79 In sheep, more pregnancies were produced from SCNT blastocysts derived from in vivo matured oocyte cytoplasts compared with SCNT blastocysts from in vitro matured oocyte cytoplasts.80 In contrast, there was no difference in pregnancy rate following transfer of transgenic embryos at the morula or blastocyst stages based on whether they were from in vivo versus in vitro maturation conditions.79 However, for transgenic blastocysts developed from bovine oocytes matured in vivo versus those matured in vitro, there was a difference in the sex ratio of the calves, with a higher percentage of males born in the in vitro matured group compared with the in vivo fertilized group.79 Interestingly, the mean birthweights of male or female transgenic calves resulting from the transfer of blastocysts from oocytes matured in vitro were significantly greater than those for male or female calves produced from blastocysts from oocytes matured in vivo.79 In addition, 48% of calves from in vitro matured oocytes were 45 kg or more at birth, whereas only 19% of calves from in vivo produced oocytes were 45 kg or more at birth. Finally, female calves produced from in vitro matured oocytes were significantly heavier than male calves produced from in vivo matured oocytes. Taken together, observations in both cattle and sheep support the conclusion that differences between in vitro and in vivo oocyte maturation conditions plays an important role in determining the occurrence of at least type IV AOS and possibly other AOS subtypes as well. Other factors that can affect oocyte developmental competence include body condition score and diet.81 Oocytes recovered from heifers in moderate body condition that were fed diets containing high levels of metabolizable energy showed reduced yields of IVP blastocysts whereas oocytes from heifer that were in low body condition on the same high dietary energy levels showed improved in vitro blastocyst development.81 Oocytes from heifers on diets designed to generate elevated levels of plasma ammonia demonstrated reduced in vitro developmental competence (cleavage to 2-cell and blastocyst stages) compared with controls.82 In sheep, embryos produced in vitro from oocytes exposed in vivo to elevated dietary urea nitrogen experienced reduced pregnancy rates following transfer.68 Taken together, these observations provide insight into possible mechanisms accounting for early observations that diets high in protein are associated with reduced fertility in dairy cattle83 and also provide insight into potential mechanisms contributing to the occurrence of type I AOS. In cattle, embryos are typically transferred on day 7 of gestation at the blastocyst stage of development. The greatest degree of pregnancy loss in cattle occurs within the first 2–3 weeks of gestation, with the majority of losses occurring between days 8 and 16.84 Compared with AI or the transfer of in vivo produced embryos, IVP embryos can experience even greater rates of early embryonic death; however, this can be influenced by the quality of the embryo transferred. For example, survival rates for bovine embryos of quality grade 1 were the same whether derived from in vivo or in vitro production systems; however, for embryos of quality grade 2, survival rates were lower if from in vitro production systems.45 Pregnancy rates and calving rates following the transfer of IVP embryos vary both across and within laboratories, although they can be lower than those seen following AI or the transfer of in vivo produced embryos.54,55,85–88 Other factors such as embryo evaluator89 and recipient characteristics86 can also influence the successful establishment and maintenance of pregnancy following transfer of nonfrozen in vivo and IVP bovine embryos. Preimplantation-stage bovine embryos produced in vitro differ in morphology compared with in vivo produced embryos. If produced in serum-containing medium, bovine embryos appear darker, have a less-organized inner cell mass, and exhibit a less complete compaction.76,78 IVP embryos, regardless of whether they are produced in serum-containing or serum-free media, have greater numbers of lipid droplets, more immature mitochondria, and do not exhibit a comparable increase in mitochondrial density as they transition from the morula to blastocyst stage compared with in vivo embryos.53,90–94 Total cell number per blastocyst and allocation of blastomeres to the inner cell mass of blastocysts also differs between in vitro and in vivo production systems. IVP blastocysts demonstrate fewer total cells and a smaller proportion of cells allocated to the inner cell mass.94,95 Differences also exist in the extent of abnormal ploidy (chromosome number) among blastomeres of embryos produced in vivo compared with their in vitro counterparts.96 Whereas only 20–25% of blastocysts from in vivo production systems demonstrate mixoploidy, approximately 45–70% of IVP blastocysts were determined to be mixoploid with the incidence dependent on the method used to detect chromosome number.97,98 Although embryos carrying increased proportions of mixoploidy can successfully establish and maintain pregnancy,96 severe increases in the number of mixoploid blastomeres are not conducive to embryo survival.99 Early comparisons of gene expression for bovine preimplantation embryos produced in vivo and in vitro demonstrated that expression of both imprinted and nonimprinted genes can be affected by in vitro embryo production.100–109 Additional support for the hypothesis that the competence of blastocysts to establish pregnancy is associated with expression of specific gene sets was provided by investigators who analyzed mRNA expression from biopsies of blastocysts taken prior to embryo transfer and with their subsequent post-transfer events. Blastocysts that were not destined to establish pregnancy were found to express elevated levels of the implantation inhibitor CD9, as well as the inflammatory cytokine tumor necrosis factor (TNF) and mRNAs associated with carbohydrate metabolism.110 Conversely, blastocysts whose transfer resulted in delivery of a calf demonstrated increased expression of a number of specific mRNAs, including some associated with implantation (COX2, CDX2, PLAC8) and differentiation (BMP15). These observations were consistent with an earlier report correlating mRNA expression profiles with post-transfer pregnancy success of demi-embryo pairs produced by splitting blastocysts obtained from superovulated donor cows.111 These investigators also noted elevated expression of PLAC8 in demi-embryos whose twinned demi-blastocyst was associated with calf delivery following transfer. To address the question of when mRNA profiles predictive of developmental competence could be determined during development, Held et al.112 assessed the mRNA expression pattern from isolated blastomeres from 2-cell IVP bovine embryos with the developmental competence of their sister blastomeres. These investigators determined that sister blastomeres destined to develop to the blastocyst stage expressed elevated levels of mRNAs associated with oxidative stress responses, antioxidant activity, and oxidative phosphorylation.112 Taken together, these observations suggest that mRNA signatures of embryos at various stages of development can be associated with both pre- and post-transfer developmental outcome. Furthermore, they are consistent with the hypothesis that, in the future, specific mRNA signatures for embryos destined to exhibit specific AOS subtypes following embryo transfer may also be identifiable. This, in turn, will support development of pre-transfer embryo screening procedures or refinement of in vitro embryo production methods to eliminate the occurrence of AOS. For example, inclusion of specific supplements to medium used for production of bovine embryos in vitro, including insulin-like growth factor (IGF)-1, colony-stimulating factor (CSF)-2 and hyaluronan, have been demonstrated to improve pregnancy rate after transfer.113 Interestingly, addition of CSF-2 into culture medium eliminated the occurrence of embryonic loss prior to day 35 of gestation (type I AOS); however, the number of observations in this study was somewhat limited.113 It is estimated that moderate-producing dairy cows experience about a 40% embryonic and fetal mortality rate; about 70–80% of this loss is sustained between days 8 and 16 of gestation.114 Maternal recognition of pregnancy occurs around day 15–16 of gestation in cattle, and requires adequate expression of interferon (IFN)-tau from the developing conceptus to prevent the luteolytic cascade.115,116 Higher rates of pregnancy loss occur for conceptuses around the peri-implantation period following the transfer of IVP embryos.53,105,117,118 Similarly, significant embryonic loss has been reported in the 2-week period following the transfer of IVP and SCNT embryos, although a portion of this loss could be attributed to difficulties in recovering these conceptuses. For example, recovery rates of 51.2% at day 12 and 33–38% at day 14 were found following the transfer of IVP embryos.105,119 At day 14, 75% of IVP embryos transferred were lost, compared with 85–93% for SCNT embryos.118 At day 25 of gestation, only 19% and 15% of conceptuses derived from IVP and cloned embryos, respectively, were recovered compared with 67% of conceptuses produced by AI.120 In addition to lower recovery rates, conceptuses derived from IVP embryos also exhibited some morphological differences from those derived in vivo. For conceptuses derived from IVP embryos that were recovered on day 17 of gestation, there was an increased incidence of fragmentation and/or degeneration compared with in vivo-derived controls.45 In addition, IVP conceptuses are also longer than in vivo-derived conceptuses both at day 12 and day 17 of gestation.105,117 In contrast, other investigators found no statistical difference in the recovery rate of intact conceptuses on day 16 between IVP and in vivo-derived groups; however, they did report that there were more total structures or degenerated nonhatched embryos recovered in the IVP group compared with in vivo-derived controls.104 In that study, conceptuses derived from IVP embryos and recovered on day 16 of gestation had smaller embryonic disks and tended to have shorter trophoblastic lengths compared with in vivo-derived controls. Differences in conceptus length between studies could be attributed to differences in in vitro conditions used to produce the embryos as well as to the timing of recovery relative to maternal recognition of pregnancy. Compared with conceptuses derived from the transfer of IVP embryos, 20% fewer conceptuses from SCNT embryos had an embryonic disk at recovery on day 17.121 Recovery rate is generally higher when an embryonic disk is detected with no signs of conceptus degeneration.119 Culture conditions can influence the presence of an embryonic disk in developing conceptuses; one study reported a higher percentage of embryonic disks present when KSOM medium (72%) was utilized compared with SOF medium (46%).119 Peri-implantation conceptuses derived from IVP or SCNT embryos may have different developmental and ultrastructural properties.118 Both IVP and SCNT conceptuses were developmentally delayed at day 14 and day 21 compared with the expected developmental timeline described for in vivo conceptuses.118 When comparing conceptuses from IVP- and SCNT-derived embryos, SCNT conceptuses at both days 14 and 21 of gestation were shorter in length and displayed more ultrastructural deficiencies at both time points compared with IVP conceptuses.118 For example, SCNT conceptuses exhibited a greater occurrence of apoptosis, more vacuoles, fewer mitochondria in the hypoblast, disintegration of the basement membrane between the hypoblast and epiblast, less defined tight junctions between trophectoderm cells, fewer desmosomes, and fewer polyribosomes. Differences in gene expression have been observed between conceptuses derived from the transfer of IVP or in vivo-produced embryos.104 For example, in vivo control embryos showed a temporal reduction in transcriptional activity from day 7 to day 16 that was not seen during this period in IVP embryos. Furthermore, on day 16 of gestation conceptuses from IVP-derived embryos had greater expression of IGF-1R mRNA transcript compared with in vivo controls.104 While day 7 IVP blastocysts had a lower relative level of IFN-tau transcript compared with in vivo-produced controls, developmentally delayed day 16 IVP conceptuses had greater content of IFN-tau mRNA.104 Compared with IVP conceptuses, conceptuses derived from the transfer of SCNT embryos lacked expression of NANOG and FGF4, and overexpressed IFN-tau mRNA at day 17 of gestation.121 Using a custom microarray, 47 genes were identified as differentially expressed at day 17 between conceptuses from SCNT and IVP embryos. Furthermore, expression of genes involved in trophoblast proliferation (Mash2), differentiation (Hand1), and trophoblast function (PAG-9) were altered in SCNT conceptuses at day 17 compared with conceptuses from either IVP or AI.122 At day 25 of gestation, conceptuses derived from IVP or NT embryos had a greater expression of IGF-1 mRNA compared with AI controls. In addition, conceptuses derived from SCNT embryos had greater expression of IGF-2 mRNA than IVP-derived conceptuses.120 Interestingly, gene expression profiles from uterine endometria of pregnancies derived from SCNT and IVP embryos differ.123 These observations support the suggestion that during the peri-implantation period, abnormal embryo–maternal communication could be responsible for the altered phenotype in cloned animals.123 In effect, altered expression of genes vital to normal trophoblast differentiation and subsequent placental development associated with IVP and SCNT may be factors contributing to increased pregnancy losses and other abnormalities observed later in development. Development of fetuses following the transfer of IVP or SCNT embryos is associated with increased fetal mortality and a multitude of fetal abnormalities. Following the period of embryonic loss in cattle, fetuses derived from IVP or SCNT embryos experience higher rates of gestational loss compared with those produced in vivo (Figure 67.4). A large-scale study analyzing results from over 30 datasets on IVP and SCNT reported that after adjusting for effects such as season, parity, and sex of calf, fetal losses were higher for both IVP and SCNT embryos compared with AI or embryo transfer pregnancies.55 Furthermore, IVP and SCNT calves experienced longer gestation lengths, higher birthweights, and increased incidences of dystocia and perinatal losses compared with AI or embryo transfer calves. Figure 67.4 Relative timing of pregnancy losses following the transfer of bovine embryos produced in vivo, in vitro (IVP), or by somatic cell nuclear transfer (SCNT). Higher rates of pregnancy loss following the transfer of IVP embryos are seen during the late embryonic period (day 30) and early fetal period up to day 90 of gestation compared with in vivo controls.59 While the rate of pregnancy loss from approximately 2 months of gestation to term is less than 10% in recipients carrying in vivo-produced embryos,124–126 this loss can be two to three times greater in recipients carrying IVP embryos.7,54,127,128 IVP pregnancies experience embryonic and fetal losses particularly between days 30 and 65 of gestation, with the majority of the losses occurring between days 30 and 44.128 By comparison, pregnancies derived from embryos produced in vivo had lower rates of loss that were distributed more evenly between days 30 and 90.128 Furthermore, pregnancy rate at day 60 of gestation was 42% lower in recipients with grade 1 IVP embryos compared to those with grade 1 in vivo-produced embryos.45 Pregnancy rate at day 50–60 of gestation following the transfer of grade 1 fresh IVP embryos (59%) was significantly lower compared with that of grade 1 fresh in vivo-produced embryos (76%).54 However, based on data from a large-scale study, pregnancy rate between days 90 and 150 of gestation was similar for both fresh and frozen IVP embryos (43.5%).129 The occurrence of early fetal mortality is even greater following the transfer of cloned (NT and SCNT) embryos. Losses of 50–100% of pregnancies from cloned embryos can occur between days 30 and 60 of gestation, compared with a 2–10% loss of pregnancies from natural service that normally occurs during this period.15,20,62,130 A second period of high pregnancy loss occurs during the second trimester of pregnancy for cloned pregnancies.15,62,131 In many of these cases, while few gross abnormalities may be noted in the aborted fetuses, the placentas are often grossly abnormal.15,62,131 Cloned pregnancies may also be subjected to greater losses during the third trimester, often characterized by placental abnormalities including hydrallantois, reduced placentome number, and edema in the placental membranes.15,62 Based on a nationwide survey in Japan on the survival of embryos and calves derived from SCNT from 1998 to 2007, the survival rate of SCNT embryos and calves had not improved during that 10-year period.132 Pregnancy rates at day 30 following the transfer of SCNT embryos ranged from 21.6 to 32.9% and were lower compared with pregnancy rates following the transfer of in vivo-derived embryos (50–52%) and IVP embryos (37–46%).132 In addition to an increased incidence of fetal mortality, fetuses derived from IVP and SCNT embryos exhibit a variety of morphological abnormalities during early gestation. Compared with AI controls, SCNT and IVP fetuses at day 80 of gestation had increased body weight, liver weight, and thorax circumference.133 Furthermore, these investigators found that the ratio of crown–rump length to thorax circumference was a defining morphological characteristic for the phenotype of disproportionate fetal overgrowth. In cloned fetuses, decreased crown–rump length and/or fetal heart beat were predictive ultrasound markers for fetal death that occurred in about 27% of clones that died prior to day 90 of gestation.49 These investigators noted that, based on ultrasound assessments after day 100 of gestation, risk factors for loss of SCNT-derived fetuses included placental edema, hydrops, and increased abdominal circumference. The frequency of congenital malformations, which include hydrallantois and abnormal limbs, is increased in calves from IVP embryos (3.2%) compared with AI controls (0.7%).129 At day 90 of gestation, IVP pregnancies were associated with increased fetal crown–rump lengths and organ weights compared with in vivo controls.134 At day 180 of gestation, IVP pregnancies were associated with greater uterine, placental and fetal weights compared with in vivo controls. Fetal weight, fetal liver, fetal heart, and placentome weight were all correlated and were significantly greater in IVP pregnancies.134 At day 222 of gestation, fetuses from IVP embryos were 17% larger than in vivo-produced controls and exhibited significant increases in fetal body weight, heart girth, heart weight, and long-bone length.45 Furthermore, fetuses from IVP embryos exhibited no difference in gross visceral organ weight but had smaller skeletal muscle measurements per kilogram body weight compared with fetuses from the in vivo-derived group, suggesting that IVP fetuses develop disproportionately to their body weight. Histologically, IVP fetuses at day 222 of gestation exhibited a ratio of secondary to primary skeletal muscle fiber number that was significantly greater than that for in vivo-derived controls. Similarly, the proportional volume of tissue present between myofibrils was also greater in muscle of IVP fetuses compared with controls.135 Expression of myostatin mRNA was decreased in the skeletal muscle of fetuses from the IVP group, while expression of glyceraldehyde 3-phosphate dehydrogenase (GAPDH) tended to be increased compared with the in vivo-derived group. These observations are consistent with the observed changes in muscle fiber ratios since myostatin is an inhibitor of muscle fiber development and GAPDH is a key enzyme in glycolysis, a pathway that is heavily used by secondary muscle fibers.135 Perinatal mortality rates of 8.6–15.6% from IVP calves at birth or shortly after birth were reported in recipient heifers, and were as high as 17.9% for recipient cows.7,54,129 In contrast, perinatal mortality rates in AI calves were approximately 6.2–9.0% for in vivo embryo transfer pregnancies.22,129 Some studies have reported an increased gestation length for IVP fetuses,129 whereas others have not.44 The incidence of stillbirth and perinatal mortality within the first 48 hours after calving was increased in IVP calves compared with in vivo-derived controls but was not due to altered gestation length.136 Stillbirth and postnatal mortality were also increased in SCNT pregnancies. Only about 70% of cloned cattle survive the perinatal period and these losses are generally sustained within the first week of postnatal life (reviewed in ref. 48). Based on the 2012 national survey by the Japanese Ministry of Agriculture, of 594 cloned calves born in Japan between 1998 and 2007, 14.8% were stillborn and 95% were dead within 24 hours after parturition.137 The calving rate for SCNT pregnancies (5.1–12.6%) was also lower than for in vivo embryo transfer (23.9–34.8%) and for IVP (20.2–22.3%) pregnancies.132 Based on data from 5 years of commercial cloning practice in the United States, Argentina and Brazil,49 of 3374 SCNT embryos transferred, 41% were alive at day 30 of gestation but only 9% of the total embryos transferred resulted in live births. Of these 388 live SCNT calves, about 42% died between parturition and 150 days of life.49 The efficiency of cell lines for successful production of viable SCNT pregnancies ranged from 0 to 45%, with about 24% of cell lines failing to produce live calves.49 The most conspicuous abnormalities seen in the perinatal period following the transfer of IVP embryos is the occurrence of high birthweight calves in association with a broader distribution of birthweight compared with in vivo controls. High rates of perinatal pregnancy loss associated with IVP pregnancies can be largely attributed to dystocia occurring as a result of large-sized fetuses.44,59 Dystocia can have long-term effects on recipient animal health, production, and future reproductive performance.59 High birthweight in association with IVP of transferred embryos has been reported for several Bos taurus breeds including Holstein, Angus, Simmental, and Japanese Black.55,129,136,138,139 Transfer of IVP-derived embryos in Bos indicus Gyr cattle also resulted in higher birthweights than AI controls, with particular effect observed for male calves.140 Interestingly, IVP calves demonstrated not only higher birthweights but also elevated plasma fructose concentrations after birth compared with in vivo controls.134 These factors appeared to be associated with metabolic distress in the IVP calves.134 Common morphological abnormalities reported following the transfer of SCNT embryos included enlarged umbilical cord, respiratory problems, prolonged recumbency, and contracted flexor tendons.49 In addition, cloned calves demonstrated other abnormalities at birth including hypothermia, hypoglycemia, severe metabolic acidosis, and hypoxia, although these parameters were not significantly correlated with birthweight. Because plasma insulin concentrations were significantly increased and plasma glucagon concentrations correlated with birthweight, aberrant energy availability and energy utilization in utero were proposed to induce conditions similar to that seen with human gestational diabetes.141 At birth, cloned calves often exhibit respiratory distress syndrome142 and, in some cases, exhibited left-sided cardiopathy with ventricular dilation and pulmonary hypertension.142 In addition, calves that appeared abnormal at birth also exhibited an edematous placenta.142 Other studies have reported enlarged umbilical vessels and varying degrees of edema as well as illnesses such as rumenitis, abomasitis, and coccidiosis at birth.15,20,52,64,143 Mean placentome number was also lower and mean placentome weight higher in placentas of cloned calves compared with IVP controls.52 Fetuses and calves from IVP or SCNT embryos exhibit a right-skewed weight distribution compared with AI or in vivo-produced embryo transfer controls.52,54,55,144 Cloned calves were 20% larger at birth compared with in vivo-produced embryo transfer or AI calves, and exhibited greater variation in birthweight.33 Interestingly, cloned calves derived from oocytes matured in vitro had a significantly higher birthweight than those cloned calves derived from oocytes matured in vivo. In fact, the mean birthweight of the female in vitro-derived calves was significantly higher than that of the in vivo-derived male calves.79 The proportion of male offspring resulting from the transfer of IVP embryos has been reported to be increased from the expected 1 : 1 ratio compared with AI controls where a normal sex ratio was maintained.104,129,140 In contrast, other studies have not reported a difference in the proportion of males born following the transfer of IVP embryos.45,54,145 Fetuses derived from IVP embryos may also differ in gene and protein expression compared with those produced in vivo. At day 90 of gestation, IVP pregnancies were associated with lower fetal d-glucose concentrations than in vivo controls.128 Expression of IGF-2 mRNA was twofold greater in day 63 liver tissue but decreased in skeletal muscle tissue of fetuses derived from IVP embryos compared with that of in vivo-derived controls.146 Furthermore, skeletal muscle tissue from fetuses at day 222 of gestation derived from the transfer of IVP embryos had lower expression of myostatin mRNA and tended to have increased expression of GAPDH mRNA compared with in vivo controls.147 In contrast, despite differences in fetal and placental morphology at day 90 or day 180 of gestation between pregnancies derived from IVP or in vivo produced embryos, no significant difference in levels of mRNA expression were detected in fetal liver and placental tissues from these pregnancies when a 13 000 oligonucleotide microarray was used for analysis.148 Because these results contrasted with data from earlier studies, Jiang et al.148 proposed that abnormal gene expression could be stage and tissue specific. Alternatively, the limited number of observations used within each treatment/stage subgroup, coupled with a limited size expression array, may have precluded identification of differences in mRNA expression. In addition to fetal abnormalities, the production of calves following the transfer of IVP or SCNT embryos is associated with defects in placental development and vasculature. Placentas of IVP or SCNT pregnancies can exhibit differences in morphology, function, gene expression, hormone production, or protein production compared with placentas from in vivo-derived embryos or AI pregnancies.15,51,52,59,62,149 Perturbations in placentation and placental function could affect growth and development of those fetuses produced from in vitro techniques. Abnormalities in placental development are even more pronounced in pregnancies from SCNT embryos; in fact, the majority of pregnancy loss in SCNT pregnancies is attributed to placental deficiencies.62,142 In contrast, in normal (in vivo-derived) pregnancies, the majority of losses are due to fetal abnormalities.54
Abnormal Offspring Syndromea
Utilization of embryo biotechnologies in cattle
Recognition of the effects of embryo manipulation on offspring development
Classification system for abnormal offspring syndrome
Characteristics associated with AOS during bovine development
Gametes
Sperm
Oocytes
Preimplantation development
Peri-implantation development
Fetal development
Placental development
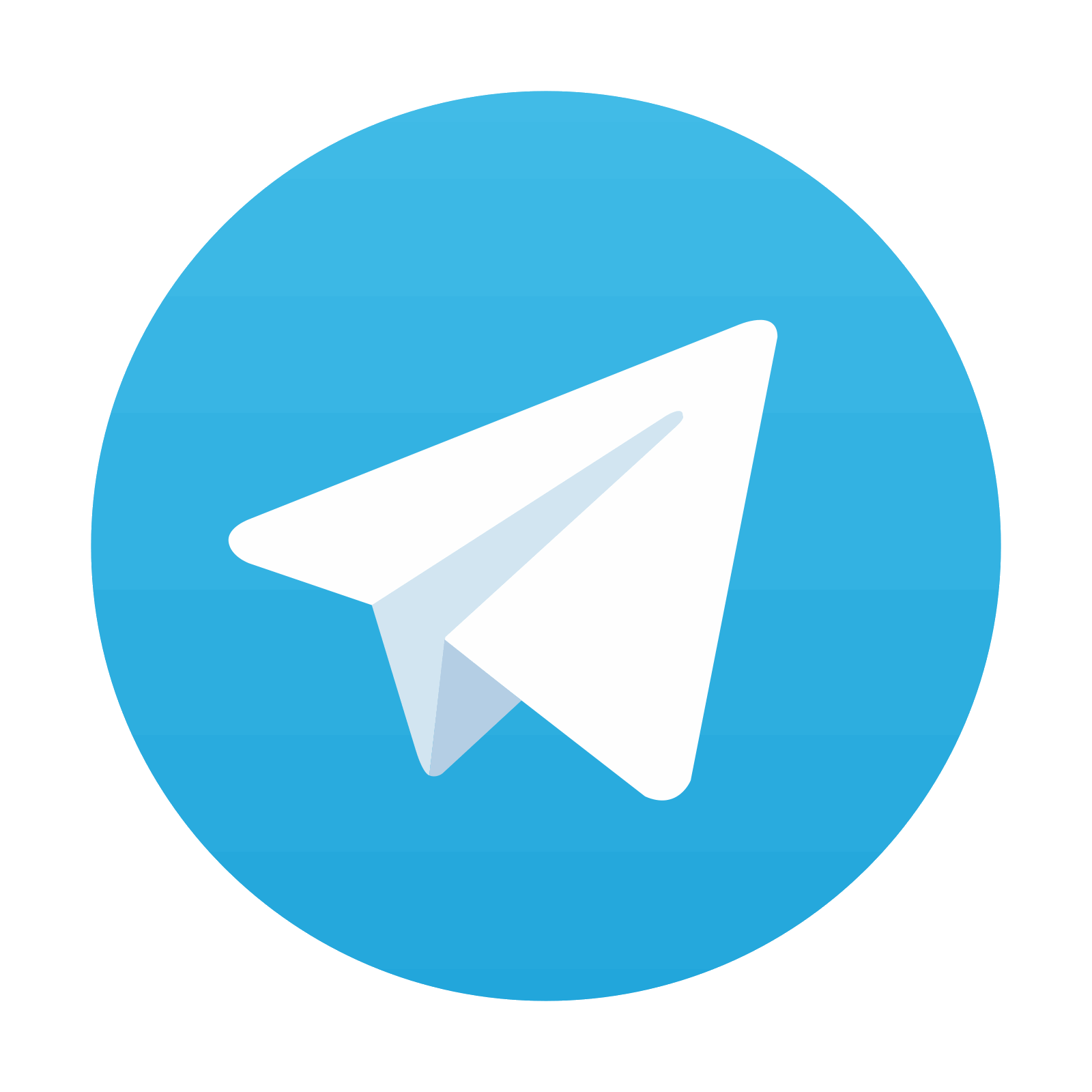
Stay updated, free articles. Join our Telegram channel
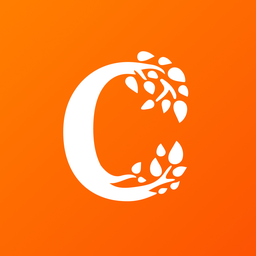
Full access? Get Clinical Tree
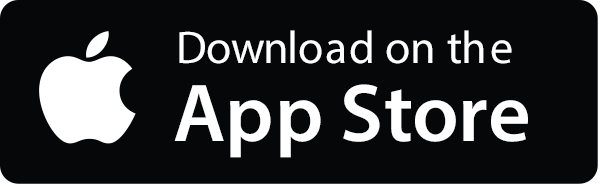
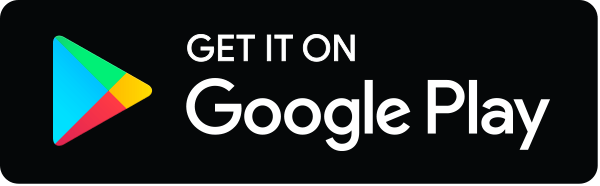