Fig. 11.1
Recording of sleep stages through EEG. Each trace is a representative recording for the indicated stage. Note the increase in voltage amplitude as NREM progresses from stage 1 to stage 3, in which SWS with typical high-amplitude/low-frequency delta waves appear. Note also the similarity between the wake and REM sleep EEG. Source: Horne (1988)
While EEG has remained the definitive method of measuring sleep for nearly half a century, other methods of sleep measurement capitalize on physiological correlates of sleep. The reduction or near absence of locomotor activity provides an easily measurable marker of sleep. Locomotor activity can be measured using a range of techniques. Accelerometers and global positioning satellite monitoring can be attached to animals by collars around the neck or legs, and they monitor locomotor activity and range of movement over time in an open environment. Locomotor activity in animals in their natural environment can be monitored for long durations across far distances. For example, in nocturnal owl monkeys, long-term locomotor activity was recorded for up to 18 months (Fernandez-Duque et al. 2010). In a confined environment, locomotor activity can be measured more precisely using a noninvasive infrared grid that counts beam breaks. Recently, video tracking of animals has become attractive, as hardware and software advances have enabled the accurate automated scoring of not only wake and sleep but the characterization of NREM and REM sleep stages in rodents (Fisher et al. 2012; McShane et al. 2012). Video monitoring allows the tracking of multiple animals in the same living environment and for the screening of many animals to search for interesting sleep phenotypes. In Drosophila, dozens of animals can be simultaneously tracked in test tubelike actimeters. The high-throughput measurement of sleep combined with the powerful fruit fly molecular genetics greatly accelerates the identification of genes involved in the regulation of sleep (Cirelli 2009; Sehgal and Mignot 2011).
In humans, actigraphy is frequently used to monitor activity levels, and advanced algorithms have been developed to identify periods of sleep (Sadeh et al. 1994; de Souza et al. 2003). Wristwatch-like activity monitors can record up to weeks of activity. As opposed to EEG, determining sleep from actigraphy is less accurate, as movement does not always correlate with the absence of sleep and lack of movement with its presence. However, actigraphy has the benefit of being a simple, inexpensive method for measuring sleep for long durations with no maintenance.
11.3 Timing of Sleep
Under natural conditions each species has a typical 24-h temporal distribution of wake and sleep, which determines a “24-h temporal niche” for that species. Humans are diurnal and display nocturnal sleep, whereas nocturnal species typically sleep during the day. What determines the temporal organization of sleep and wake? In other words, why does a songbird sleep during the night and an owl during the day?
We have all experienced how the pressure to fall asleep increases as we stay awake. Sleep pressure increases as a function of wake time; in contrast, sleep pressure decreases with sleep time, and the longer sleep lasts, the more difficult it is to sustain it. This regulatory process is known as the homeostatic or sleep–wake-dependent regulatory process of sleep (Borbely 1982; Borbely et al. 2001). Figure 11.2b schematizes the dependence of sleep pressure on wake and sleep times. On a typical day a human adult wakes up in the morning (7:00) with little or no sleep pressure. As the individual progresses through the day, sleep pressure increases steadily with the duration of wake, reaching a daily wake-dependent threshold at the usual bedtime (23:00). When he/she falls asleep and as sleep takes place during the night (black areas in the plot), sleep pressure decreases to once again reach a sleep-dependent minimum. Figure 11.2a also shows that interfering with the subject’s habitual bedtime—working late into the night or a Saturday night party—will allow the “sleep homeostat” to increase sleep pressure beyond normal daily values (dotted line). Thus, the homeostatic process of sleep keeps track of both sleep and wake hours and will prevent excessive sleep deprivation when the sleep pressure is so high that sleep must occur; conversely, it will allow wake after a given duration of sufficient sleep.
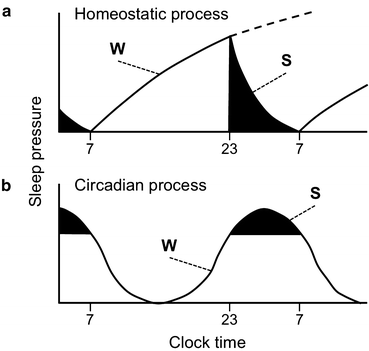
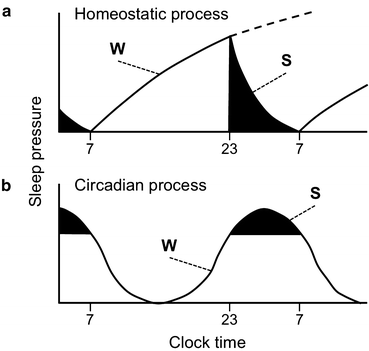
Fig. 11.2
Sleep pressure in normal healthy humans is regulated by homeostatic (a) and circadian (b) sleep regulatory processes. W, wake; S, sleep. Black regions indicate sleep time, which occurs between 23:00 and 7:00. The dotted line on (a) depicts how sleep pressure increases through the homeostatic mechanism if there is sleep deprivation
The homeostatic regulatory process determines the need for sleep and its intensity when sleep begins (see below), but it does not determine the phase of sleep within the 24-h day, that is, at what time of the day sleep and wake should take place. How do diurnal and nocturnal animals determine their respective nighttime and daytime sleep bouts? The second regulatory process of sleep, known as the circadian process, regulates the time of the day sleep will likely occur, regardless of the sleep or wake history. In other words, sleep pressure oscillates with a 24-h period and this oscillation, with a peak of sleep propensity at a specific time of the day, represents an output of the circadian system. Figure 11.2b illustrates the circadian process of sleep as an oscillation of sleep pressure. In humans whose circadian system is entrained to the solar light–dark (LD) cycle, the circadian peak of sleep propensity occurs around 3 AM, which explains the high sleep propensity we feel at this time when we try to stay up all night. Interestingly, the peak of circadian sleep pressure does not occur at the same time as the peak of homeostatic sleep pressure; instead, as the homeostatic drive to sleep wanes, the circadian drive to sleep reaches its peak. Because overall propensity to stay asleep depends on the cumulative contributions of the homeostatic and circadian processes, this paradoxical mismatch between the homeostatic and circadian peaks of sleep pressure allows us to have an extended and consolidated bout of nocturnal sleep (Dijk and Czeisler 1994).
11.4 Uncovering the Circadian Nature of Sleep
What is the evidence that this peak of sleep pressure in the second half of the night represents a circadian rhythm? The golden standard for the demonstration of the circadian nature of a rhythm is its persistence under constant laboratory conditions, which reveals the existence of the circadian clock that generates the rhythm. Studies in the 1960s showed that indeed the human rest–activity cycle persists with a period of circa 24 h in humans (Aschoff 1965), and free-running rhythms of EEG-detected sleep stages were later confirmed (Czeisler et al. 1980b). Furthermore, several laboratories have reported clear circadian rhythms of polysomnographic sleep in nocturnal rodents and nonhuman primates under free-running conditions (see Mistlberger 2005 for an in-depth review). In fact, the circadian regulation of sleep appears to be a common feature in virtually all species studied so far, including invertebrates (Sehgal and Mignot 2011).
Interestingly, the temporal organization of human sleep under constant laboratory conditions is rather different from that under a normal 24-h LD cycle. First, the self-selected circadian time to go to bed coincides with the minimum of core body temperature (CBT) (Czeisler et al. 1980b; Zulley et al. 1981) whereas, sleep onset under a 24-h LD cycle occurs typically at the end of the evening (2300 in Fig. 11.2), after several hours of waking due to exposure to daytime light and several hours before the temperature minimum. Second, humans under constant environmental conditions often adopt rest–activity—and associated sleep–wake—cycles with periods that are much longer than 24 h. Under these conditions, the regulation of the rest–activity cycle is out of synchrony with the circadian regulation of several physiological processes, leading to a state known as spontaneous internal desynchrony. Although at first glance this appears to challenge the notion of a robust circadian regulation of sleep, careful analysis of the timing of sleep under spontaneous internal desynchrony has revealed some of the key features of its circadian regulation. First, most of the spontaneous sleep episodes in internally desynchronized subjects are initiated during the circadian trough of temperature. In other words, although they freely choose their bedtime, there is a clear propensity for them to fall asleep at a very specific circadian time. Second, the duration of each sleep episode depends on the circadian time at which sleep started. The longest sleep bouts are those started during the peak of rhythmic CBT, and sleep episodes that start at the rising phase of the CBT rhythm are shorter. This rhythm of sleep propensity is also manifested during the wake episodes as increased alertness at circadian times when the CBT is at its peak (Czeisler et al. 1980b).
Other experimental protocols have revealed circadian rhythmicity in sleep propensity. One of these protocols displaces sleep to times in which sleep typically does not take place. By extending the habitual wake time, the sleep onset can be forced to occur at different circadian times. These sleep displacement protocols have demonstrated that sleep can be started at any circadian time as long as the wake time before is sufficiently long. Similar to sleep under spontaneous internal desynchrony, the circadian regulation of sleep in displacement protocols is evidenced by longer sleep episodes when they are started at the circadian peak of CBT as well as by the increased self-rated sleepiness at specific circadian times (Akerstedt and Gillberg 1981; Dijk et al. 1991 and see review by Borbely et al. 2001).
A third experimental approach to uncover the circadian regulation of sleep is the forced desynchrony (FD) protocol. Nathaniel Kleitman pioneered this experimental approach in studies in which he subjected himself to rest–activity cycles with periods different from 24 h (Kleitman 1939; Kleitman and Kleitman 1953). He discovered that despite the fact that he could schedule himself to these rest–activity cycles, his rhythm of body temperature did not seem to synchronize to the artificially short or long days. Kleitman’s experiments led to the development of FD protocols as the most useful approach to unmask the circadian process of sleep regulation. In some of these studies, subjects are exposed to short sleep–wake cycles (for instance, 30 min of sleep scheduled every 90 min) for 1 or more days. Sleepiness just before and after these short sleep episodes as well as the amount and type of sleep within each scheduled sleep period are assessed (Moses et al. 1975, 1978 and see review by Borbely et al. 2001). Other studies use longer rest–activity periods that are closer to 24 h but still outside of the limits of entrainment of the circadian system. FD protocols offer several advantages to free-running and sleep displacement studies. Because the amount of sleep in a 24-h period can be maintained, FD protocols do not have the confounding effect of long-term sleep deprivation present in displaced-sleep studies. Furthermore, the strictly imposed non-24-h sleep–wake cycle assures that the circadian rhythms that do not entrain to this schedule, such as the CBT rhythm, come predictably in and out of phase with scheduled sleep. This represents an important advantage to spontaneous internal desynchrony, in which the experimenter cannot predict the misalignment between the self-selected sleep–wake cycle and physiological circadian rhythms. FD protocols have confirmed circadian rhythms of alertness and sleep propensity with peaks respectively aligned with the peak and trough of the CBT rhythm (see reviews by Borbely et al. 2001; Czeisler and Dijk 2001). Importantly, FD systematically changes the phase relationship between the homeostatic regulatory process—tracking the imposed rest–activity cycle—and the circadian regulatory process, following the endogenous circadian clock. This feature of the protocol has had remarkable value in discriminating how each of the two regulatory processes modulates specific sleep stages (see below).
11.5 Architecture of Sleep
11.5.1 NREM/REM Cycle
Young healthy adults sleep for about 8 h a night and cycle between NREM and REM sleep with a stereotypic temporal distribution. The NREM–REM cycle lasts approximately 90–100 min, with a total of 4–6 cycles per night. In general, in the healthy young adult, NREM sleep accounts for 75–90 % of sleep time (3–5 % stage I, 50–60 % stage II, and 10–20 % stage III). REM sleep accounts for 10–25 % of sleep time (Fig. 11.3). In nocturnal rodents, the sleep cycle is shorter, approximately 15 min, and sleep is typically much more fragmented, namely, short periods of sleep are interrupted by wake episodes (Borbely et al. 2001; Siegel 2005).
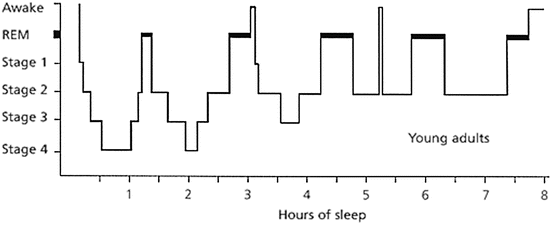
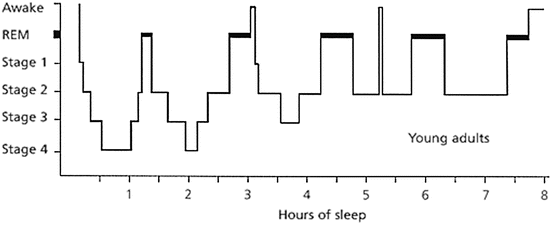
Fig. 11.3
Hypnogram of sleep architecture of a healthy young adult across an 8-h sleep period. See text for details. Source: Neubauer (1999). Note: NREM Stage 4 was redefined in 2007 by the American Association of Sleep Medicine to be included within NREM stage 3
The transition from wake to sleep begins with stage 1 NREM sleep. Following stage 1 is stage 2 NREM sleep, characterized by the presence of K-complexes and sleep spindles (Fig. 11.1). Throughout stage 2, high-voltage slow-wave activity appears and increases until meeting the criteria for stage 3 NREM sleep, also known as deep sleep or SWS. From stage 3, the individual will typically transition to stage 2 before moving into REM sleep, approximately 80–90 min after sleep onset.
The proportion of NREM (especially SWS) and REM sleep changes across a night of sleep. REM sleep duration is quite short in the first cycle, approximately 1–5 min, and increases towards the latter cycles. SWS, on the other hand, has an opposite trend and its duration decreases with successive sleep cycles. This highly conserved temporal landscape of sleep stages is known as sleep architecture. Normal sleep architecture is the result of a complex interaction between the homeostatic and circadian regulatory processes of sleep. Interestingly, although the rodent sleep cycle is shorter and sleep is much more fragmented, the regulation of sleep stages by the dual regulatory process of sleep is similar. Normal sleep architecture can be disrupted by sleep deprivation and challenges to the circadian system as well as by environmental changes that can destabilize the normal phase relationship between the sleep homeostat and the circadian system.
11.5.2 Homeostatic Sleep Pressure
Figure 11.2b clearly shows that sleep pressure increases with extended sleep deprivation. In all species studied so far, the onset of sleep after sleep deprivation shows increased SWS duration per sleep cycle and increased slow-wave activity, and the duration and intensity of SWS are proportional to the previous wake duration (reviewed by Borbely et al. 2001; Achermann and Borbely 2003). This conserved feature of the response to sleep deprivation has made of SWS a signature of the homeostatic regulatory process. Under normal conditions, homeostatic pressure is higher at the beginning of a sleep bout and decreases until the animal wakes up. SWS is thus concentrated at the beginning of the sleep bout and decreases in duration over the course of the night, matching the time course of homeostatic sleep pressure.
11.5.3 Circadian Regulation of Sleep
The circadian regulatory process times sleep to a specific phase of the LD cycle (Fig. 11.2b). Whereas SWS depends strongly on homeostatic regulation, REM sleep and the tightly associated regulation of CBT are under robust regulation by the circadian system. As the nocturnal bout of sleep progresses, homeostatic pressure, and its characteristic increased SWS, decreases. In contrast, circadian pressure increases and peaks towards 3 AM, leading to sleep cycles that get poorer in SWS but richer in REM sleep (Fig. 11.3).
The same protocols that have been key to understand the interplay between the homeostatic and circadian sleep regulatory processes in modulating sleep pressure have also been critical to unmask their influence on specific sleep states. Spontaneous internal desynchrony (Czeisler et al. 1980a, b), sleep displacement (Akerstedt and Gillberg 1981), and FD protocols (Dijk and Czeisler 1994, 1995; Dijk et al. 1999; Wyatt et al. 1999) have all shown that sleep onsets are characterized by increased SWS regardless of the circadian time at which they occur. In contrast, REM sleep propensity peaks at a specific circadian time, which coincides with the trough of the CBT rhythm, and this propensity is rather independent from previous wake time (Fig. 11.4).
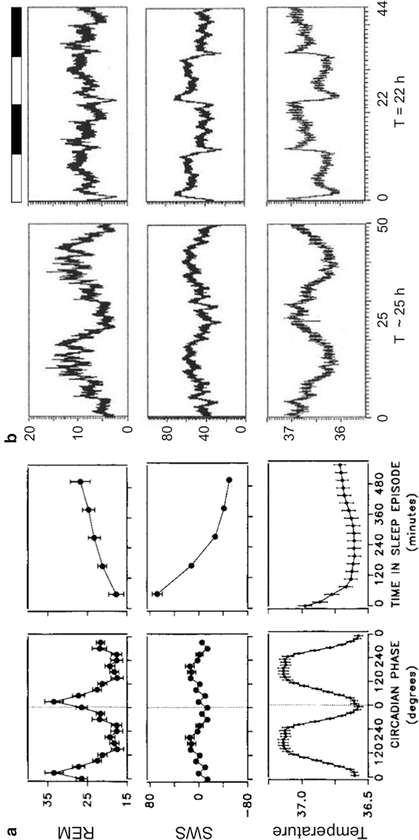
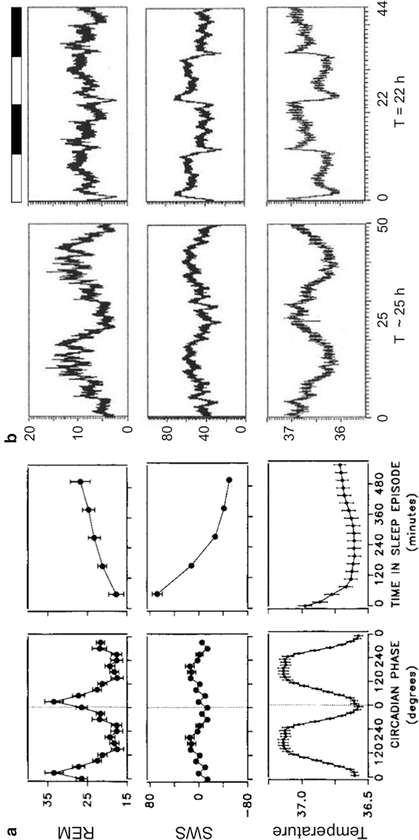
Fig. 11.4
Sleep stages are regulated by the homeostatic and circadian regulatory processes of sleep. (a) Left: in human adults under a 28-h FD protocol mean waveforms (double plotted) for REM sleep, SWS and CBT are plotted relative to the circadian period (in degrees using CBT rhythm as a phase marker). Right: the same data is plotted relative to the scheduled sleep time during the FD protocol, which lasted 9.33 h per cycle. (b) Mean waveforms for the same variables as in (a) but for a rat under a FD protocol induced by exposure to 22-h LD cycle. Left: waveforms for the three variables are plotted relative to the period of the temperature rhythm that is dissociated from the LD cycle. Right: the same data is plotted relative to the LD cycle (shown on top as black and white bars). Note that both in a and b, REM sleep has a very strong circadian regulation, independent of schedule sleep, whereas SWS depends strongly on scheduled sleep. In humans sleep is scheduled to 9.33 h every cycle and only the sleep episodes are used to generate the waveform. In rats, sleep cannot be scheduled, but the artificially short day imposes a sleep–wake cycle, and the whole cycle is used to generate the waveform. Data represent % of time in each state except for SWS in (a) which represents % slow-wave activity as deviations from the mean. Temperature is expressed in ºC. Sources: Dijk and Czeisler (1995) for (a) and Cambras et al. (2007) for (b)
Evidence in the rat indicates that the key features of sleep-stage regulation by the circadian and homeostatic processes of sleep are similar to those in humans. Rats are nocturnal and concentrate most of their sleep during the light phase. The first half of this diurnal sleep is richer in NREM sleep and SWS, whereas REM sleep becomes more abundant towards the second half of the light phase. FD can be induced in the rat by exposure to artificially short (22 h) LD cycles. Under these conditions, two rest–activity rhythms are present within the same individual. The first rhythm is entrained to the LD cycle, whereas the second is dissociated from the LD cycle and has a period longer than 24 h (~25 h) (Campuzano et al. 1998; de la Iglesia et al. 2004). Under this FD protocol, SWS oscillates in synchrony with both the 22- and 25-h bouts of rest, but REM sleep only oscillates in synchrony with the 25-h bout. As in humans under FD protocols, the peak of REM sleep propensity in the forced desynchronized rat coincides with the minimum of the CBT rhythm (Cambras et al. 2007) (Fig 11.4b).
11.6 Disruption of Sleep Architecture
Normal healthy sleep architecture can become disrupted with perturbations to the circadian system. The desynchronization of the homeostatic and circadian regulation of sleep stages, a salient feature in humans exhibiting internal circadian desynchrony, disrupts sleep architecture. Internal circadian desynchrony can result from environmental “temporal challenges” such as shift work and jet lag, but is also associated with disorders including all forms of depression, other mental disorders, and total blindness.
Shift work can include night shifts, extended work hours, or rotating shifts (variable work schedule that includes day and night shifts). Poor sleep quality is self-reported among rotating shift workers (Fido and Ghali 2008; Zverev and Misiri 2009) and night shift workers (Boivin et al. 2012 ; Juda et al. 2013; Rahman et al. 2013). Under laboratory settings, humans on a FD protocol experience less sleep efficiency and lower subjective sleep quality (O’Donnell et al. 2009). While these studies suggest circadian disruptions should result in abnormal sleep architecture in shift workers, this has yet to be investigated directly. Studies examining the rates of sleep–wake transitions in elderly (Klerman et al. 2004) and patients with obstructive sleep apnea (Bianchi et al. 2010) have documented fragmented sleep architecture in those populations.
Most psychiatric disorders show severely disrupted circadian rhythms (see reviews by Boivin 2000; Wirz-Justice 2006; McCarthy and Welsh 2012). This is particularly the case for all forms of depression, including manic-depressive illness (also known as bipolar disease) and winter depression, which are characterized by a misalignment between the timing of sleep onset and the timing of circadian rhythms such as rhythmic melatonin release (Lewy et al. 2006, 2007). REM sleep timing is among the circadian rhythms that are misaligned with sleep onset in depressive patients, which typically show an advanced REM sleep onset in their night sleep. Together, these findings have led to the “phase-advanced hypothesis,” which postulates that in depressive patients the central circadian clock is advanced relative to the onset of sleep (Wehr et al. 1979). Support for a causal role of circadian misalignment in triggering depression comes from the fact that sleep deprivation or strictly scheduled sleep has antidepressive effects (Boivin 2000; Riemann et al. 2002; Wirz-Justice 2006).
Internal desynchrony of circadian rhythms represents a chronic ailment in totally blind patients. Czeisler and colleagues showed that some blind patients retain their ability to synchronize their circadian rhythms to the LD cycle, whereas others show free-running circadian rhythms despite their self-imposed 24-h sleep–wake cycle (Czeisler et al. 1995). Later studies in animal models showed that retinal degeneration that spares intrinsically photoreceptive retinal ganglion cells preserves the ability of the master circadian pacemaker to be synchronized by the LD cycle (Schmidt et al. 2011). In totally blind patients, however, the central clock is not entrained to the LD cycle, and they typically show either an abnormal phase relationship between their circadian rhythms and the LD cycle or a free-running circadian system. Studies of their circadian rhythms in melatonin release and their temporal distribution of sleep and alertness have indicated that they behave similarly to sighted subjects under free-running conditions, namely, that increased sleepiness and decreased alertness is present during the middle of the circadian night (Lockley et al. 1999, 2007, 2008). Although EEG has not been performed in these studies, one would predict that the misalignment between the circadian and homeostatic processes in totally blind patients would lead to severe disruption of sleep architecture.
Desynchronization of sleep regulators occurs with jetlag or an abrupt phase shift of the circadian system, which disrupts the timing between the homeostatic and circadian sleep processes. In rats, an abrupt 6-h phase delay rapidly shifts the timing of the sleep–wake cycle; however, the circadian system requires several days to adjust to the phase delay. During this adjustment, the timing of locomotor activity and delta power of NREM sleep is able to quickly shift to the new schedule. The timing of REM sleep and CBT, instead, requires several days to entrain to the new schedule. As a consequence, on the days immediately after the phase shift, the peaks of REM sleep and CBT occur earlier—relative to delta power of NREM and locomotor activity—than under an unaltered 24-h LD cycle (Lee et al. 2009) (Fig. 11.5). The mistiming of REM and NREM sleep pressure results in abnormal sleep architecture, with longer durations of REM occurring in the early sleep period. One outcome of disrupted sleep regulation is the presence of REM sleep stages following wake without the typical intermediate NREM sleep. REM after wake rarely occurs in normal healthy sleep and is a characteristic of narcolepsy, a sleep disorder in which individuals are unable to maintain stable wakefulness and fall directly into REM sleep with muscle paralysis.
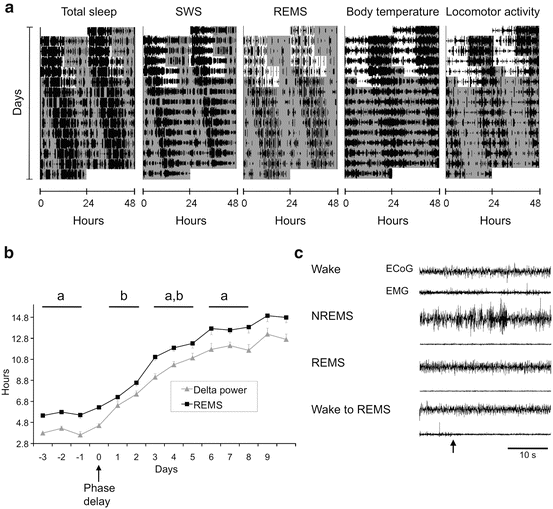
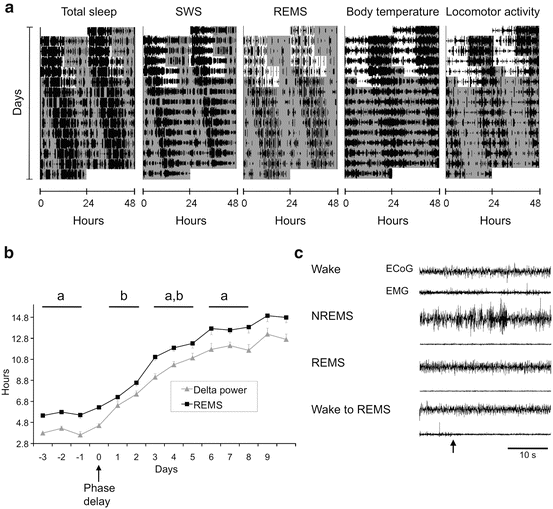
Fig. 11.5
(a) Actogram of daily total sleep, REM sleep, delta power of NREM, CBT, and locomotor activity of a rat exposed to an abrupt 6-h phase delay in the LD cycle. White and gray areas represent lights ON and OFF, respectively. (b) The daily time course of the peak of REM and delta power before and after the 6-h delay in the LD cycle (day 0) with standard error bars (n = 5). Blocks of days marked with different letters denote statistically significant differences in the peak-time difference between the REM and NREM delta power. (c) Spontaneous transition from wake to REM sleep in animal immediately after the abrupt phase delay. Representative EEG and EMG recordings are shown for each vigilance state; the wake-to-REM sleep transition (arrow) is shown in the bottom traces
11.7 Molecular Genetics of Sleep
The sleep–wake cycle and sleep architecture are directly influenced by environmental factors that can affect both homeostatic and circadian regulation. Genetic factors, on the other hand, are equally important, as they can directly affect not only the generation of specific sleep stages but also the homeostatic and circadian regulation of sleep. A review of the genes involved in sleep regulation is beyond the scope of this chapter (for recent reviews in this topic, see Jones et al. 2013; Andretic et al. 2008; Cirelli 2009; Sehgal and Mignot 2011); we will specifically focus on genes that have been directly implicated in the regulation of sleep timing that results from the homeostatic and circadian regulatory processes.
The genetics and molecular neurobiology behind the homeostatic regulation of sleep is poorly understood. In other words, genes whose products are essential for the buildup of sleep pressure during wake or of its decrease during sleep have not been identified. Sleep homeostasis is such a conserved trait across the animal phylogenetic tree that it likely serves an essential function for survival. Indeed, extended sleep deprivation leads to death in rats (Rechtschaffen et al. 1983). This may in part be why there are no single-point mutations known to abolish the homeostatic regulation of sleep. Although the evolutionarily ancestral function of sleep is unknown, it is likely that the process of sleep was co-opted for additional functions as animals evolved into more complex organisms. This notion is supported by the fact that sleep deprivation negatively impacts a wide range of biological processes, including immune response, metabolic balance, memory consolidation, and thermoregulation. Thus, sleep homeostasis may be the result of the effects of extended wake and sleep on several systems and therefore rely on the combined effects of several gene products. Nevertheless, some transmitter systems represent compelling candidates for “sleep homeostats.” Among these, adenosine, the product of the degradation of ATP and cAMP, can be produced in or released into the extracellular space and interact with specific neuronal receptors. Adenosine agonists or treatments that increase endogenous adenosine levels increase sleep and specifically stimulate SWS. Adenosine receptor antagonists, such as caffeine, inhibit sleep and SWS (Bjorness and Greene 2009). This evidence, together with the fact that adenosine levels increase with extended wake in specific brain areas, has led to the notion that adenosine levels may reflect the state of the sleep homeostat; nevertheless, several experiments have challenged this notion and it is clear that there may be other transmitter systems involved in the homeostatic buildup of sleep pressure during wake (Bjorness and Greene 2009; Sehgal and Mignot 2011).
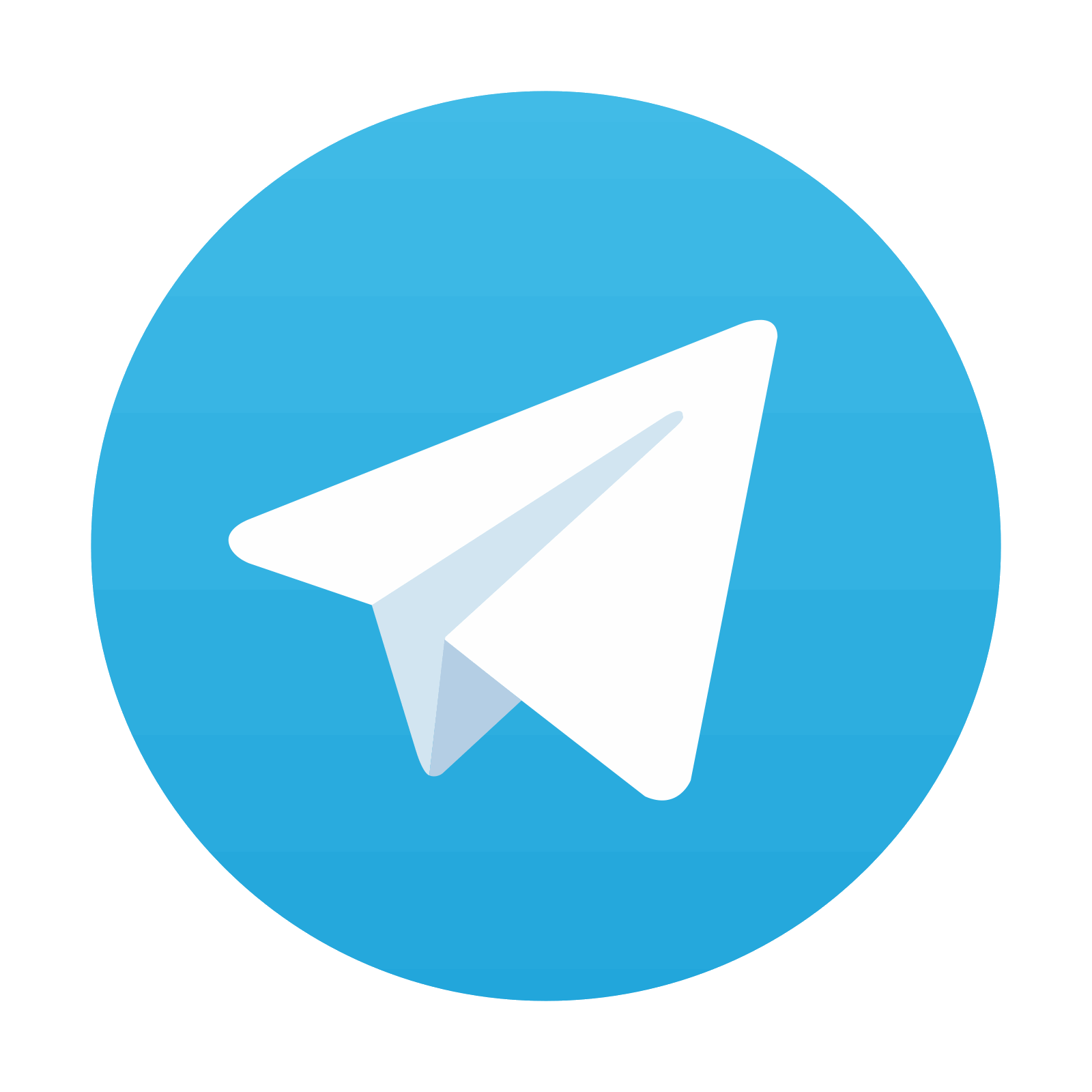
Stay updated, free articles. Join our Telegram channel
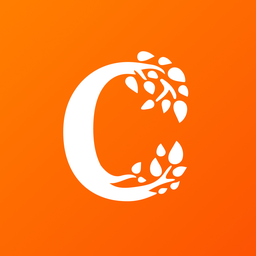
Full access? Get Clinical Tree
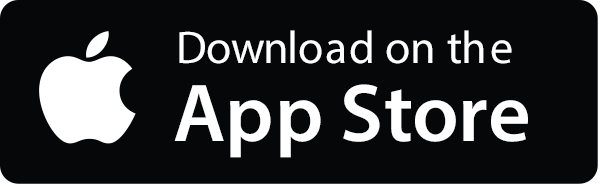
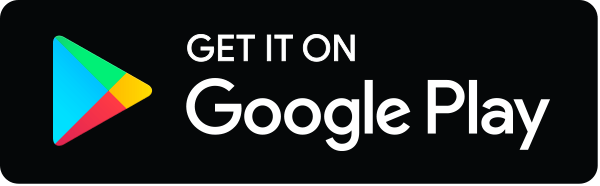