(1)
Indian Institute of Science Education and Research Pune (IISER-P), Pune, India
Abstract
Ernest Rutherford, a renowned physicist of the last century, is not only known for his discoveries but also for an often-quoted and apparently rude comment, “Only physics is science—all else is stamp collecting” [1, 2]. He was not wrong perhaps at least with reference to the mainstream biology of his times, although a conceptual revolution had already happened. Biology in its early days was nothing more than collecting information and classifying it, precisely what stamp collectors do with stamps instead of information. Physics on the other hand was able to predict things ahead of finding them based on sound theoretical foundation. For example the existence of Pluto, the ninth planet in the solar system, was predicted first and discovered eventually. So is the case with existence of some of the subatomic particles and phenomena which were predicted much before any experiments could demonstrate them. This hardly happened in biology at that time. Biology has come a long way since Rutherford’s days. The number of examples where some phenomenon in biology is predicted first and experimentally demonstrated later is on a steep rise.
Ernest Rutherford, a renowned physicist of the last century, is not only known for his discoveries but also for an often-quoted and apparently rude comment, “Only physics is science—all else is stamp collecting” [1, 2]. He was not wrong perhaps at least with reference to the mainstream biology of his times, although a conceptual revolution had already happened. Biology in its early days was nothing more than collecting information and classifying it, precisely what stamp collectors do with stamps instead of information. Physics on the other hand was able to predict things ahead of finding them based on sound theoretical foundation. For example the existence of Pluto, the ninth planet in the solar system, was predicted first and discovered eventually. So is the case with existence of some of the subatomic particles and phenomena which were predicted much before any experiments could demonstrate them. This hardly happened in biology at that time. Biology has come a long way since Rutherford’s days. The number of examples where some phenomenon in biology is predicted first and experimentally demonstrated later is on a steep rise.
One can predict things in two distinct ways. One is by finding patterns, associations, or correlations. This is not new and this is what humans have been doing since ages. For example, the association between clouds and rain can enable us to make some short-term predictions about rain. We have been doing this much before any understanding of the physical forms of water, the energetics of vaporization and condensation, etc., was known. Similarly, by observations and associations, one can predict seasonal changes in different phenomena such as flowering of plants or bird migration even without knowing any science behind it. This is a trivial form of predictability. There is no insight into causality involved in these predictions. The other is by developing an understanding of the causal connections and the ability to make good predictions by insightful understanding. This insight earmarks high-quality science. This has started happening in biology in the recent decades, taking biology out from the “stamp collection” phase to that of a full-fledged science.
There are two main reasons for this. One is our attempt to understand biology in terms of its molecules, interactions between them, and organization of these molecules into a hierarchy of structures. At this level biology becomes a complex version of physics and chemistry. All biology is in fact physics and chemistry in the sense that all laws of physics and chemistry are applicable to biological systems, and the systems work just as physical systems would work. Apart from being much more complex than systems that classical physics has handled, there is nothing nonphysical or metaphysical in biology. Therefore as the physical understanding of biological systems grows, there is better predictability in biology.
However, at the same time, a physical and chemical understanding of biology is highly inadequate. In addition to being complex physics, there is something more in biology which is not so much there in physics. An appropriate equation to represent biology would be


By history I mean the history of evolution. This is something unique to biology. There are certain parts of physics and chemistry where history does play a role. Astrophysics, geophysics, and geochemistry are good examples. But the role of history in shaping biology as a whole is not matched by any other branch of science. It is possible to study physics and chemistry with little reference to history and still get good insights into the subjects, but this is most unlikely to work in biology. Rutherford himself was perhaps aware that there is something in biology beyond physics and chemistry. Another of his quotes is almost equally famous, “When we have found how the nucleus of atoms is built up we shall have found the greatest secret of all—except life.” One needs something more than physics and chemistry to understand biology and that something is nothing other than the history and the theory of evolution.
A large number of biologists do ignore evolution and try to interpret systems as they appear today. Typically research and education in almost all branches of medicine fall in this trap. A student of medicine would typically learn his courses in anatomy, physiology, biochemistry, and the like without worrying much about how the things he has been studying came about and why they are like that. Such a view is bound to be too myopic, and biologists that ignore evolutionary history miss the point just too often. Understanding how things evolved is an essential part of understanding how and why they are so. The question why in biology is just as important as what and how, and unless the question why is addressed, biology remains nothing more than stamp collection.
The Bridge on the River Why
The question “why” can be asked and answered at a variety of levels. Why did a chameleon change its color? The why in this question can be answered in three different ways, each of the answers being “correct” in its own way. (1) The first answer could be that the chameleon changed its color because the environment changed. This is a correct answer but it only talks about the stimulus that brought about the change. (2) It can also be said that the chameleon changed its color because some pigment cells under its skin contracted and some others expanded. This is also correct as a cause of the color change, but this answers how the change was mediated. In reality this question is more of a how question than a why question. (3) At the third level we can argue that since the ability to change colors has offered selective advantages over several generations, all chameleons are able to change their colors to suit their environments. This type of reasoning tries to go at the evolutionary roots of the phenomenon and is therefore called an ultimate cause in contrast with the first two that are called proximate causes. A proximate cause is unlikely to work in the absence of an ultimate cause having worked over a long time. The ultimate cause forms the link between the stimulus and the response. For any nonchameleon the same environmental stimulus will not be able to bring about the contraction or relaxation of pigment cells under the skin, because it has not evolved that way.
Although the distinction between proximate and ultimate reasoning is made in many fields, in biology, it gets a more definitive and stable meaning. In other fields, what is ultimate at one level may become proximate at another level. In biology, these levels are more or less stable since evolution is the only and the most robust foundation for ultimate causation. So a stimulus that brings about a change and the mechanism behind the change can be considered to be at a proximate level, and the evolutionary process by which the mechanism and its responsiveness to the stimulus came into being at the ultimate level. Unfortunately almost throughout the history of biology, different sets of people have worked on the two levels of reasoning. Even today researchers working at the proximate and ultimate level have different mind-sets, and they may not even talk to each other and come out of their myopic vision, although there are some signs of the ice melting. Good biology is one where the two levels of reasoning are handled simultaneously and interactively, but so far, there are relatively few examples where the two have really been synergistic. Nevertheless, trying to bring the two together is an emerging trend in biology [3–7].
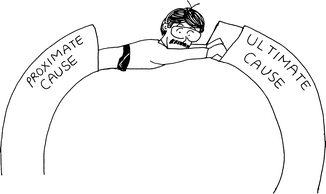
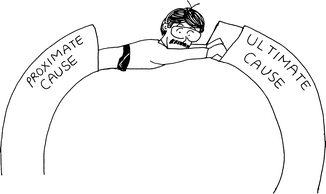
Medicine and physiology have worked mostly at the proximate level worrying little about why the systems are the way they are. Evolutionary ecologists, on the other hand, remain confined to the ultimate level most often. They would be interested in working out why animals behave the way they do, without worrying much about the mechanisms behind the behavior. Bridging the two is one of the biggest challenges of biology in near future. This book is such a bridging attempt. The attempt is limited to a specific set of questions, but the same questions are explored at both the ultimate and proximate levels simultaneously.
To make the book readable to both the proximate and ultimate biologists and to almost any science enthusiast, in Chaps.1 and 2, I will introduce both the fields separately in the context of the book. Chapter 1 introduces the way of thinking of an evolutionary biologist and is intended to provide the necessary background for a reader who may be familiar with medicine and physiology but not with evolution and ecology. Chapter 2 tries to capture the classical proximate level thinking in diabetes and related areas of medicine and physiology, and readers acquainted with ecology and evolution but not with physiology and medicine should find it useful.
Describing the concept of evolution in its full elegance is beyond the scope of this book. I have made an attempt in this chapter to introduce those concepts of evolutionary biology which we will use further in trying to understand diabetes and related disorders. An elaborate account of evolutionary concepts may no more be needed since a number of authors including Richard Dawkins, Daniel Dennet, and Matt Ridley among others have already done a great job in a series of books [8–10] readable for a nonspecialist reader. At a little more technical level, comprehensive textbooks of evolutionary biology are available including those by Futuyma [11] or Strickberger [12]. Nevertheless we will get introduced briefly to some of the key concepts of evolutionary biology that we would be using in our arguments later in this book.
The Complex Face of Adaptation
Adapting to the environmental challenges is the major if not the only force driving evolution. There is generally no dispute about this. That animals adapt to their environments was known even prior to Darwin. But throughout the history of evolutionary theory, there are serious debates about the subtleties of the nature of challenges and the nature and mechanisms of adaptations. Some debates are ongoing and yet unresolved. Some simple examples of adaptations are long known and simple to understand. Questions like why gazelle or cheetah runs fast, why carnivores have sharp canines, or why polar bears have thick fur have very simple and obvious answers that almost everyone knows. Many others look very obvious but are not in fact that straightforward. For example, flowers make nectar to attract pollinators. A simple interpretation is that pollinators get benefitted by the nectar reward, and flowers get pollinated in turn making it a simple and ideal mutualistic relationship. The reality is not as simple as it looks. What will happen if a flower decides not to make nectar? Pollinators have no way to know whether or not there is nectar in a given flower. They will discover it only by entering or probing into the flower. Once they do so, there is a good chance that the pollination job might get done, and therefore even if they do not find any nectar, the flower has nothing to lose. It is possible therefore that flowers “cheat” pollinators by not making nectar, and cheaters may do better than the honest mutualists since the cheaters get the same reward at a lesser cost. This is not a speculation alone. In a large number of species of flowering plants, some proportion of flowers are empty [13–15]. If all flowers on a given plant are empty, it is possible that pollinators will learn to avoid this plant. Therefore there is an optimum proportion of empty flowers that would keep the pollinators lured but at the same time save some cost. What this optimum proportion is depends on ecological and social factors. If the plants grow gregariously, i.e., in large numbers growing tightly together, it is more difficult for the pollinator to remember individual cheaters and shun them. In such a situation cheating is more prevalent. On the other hand, if plants grow individually isolated, they can be easily remembered and punished by pollinators, and therefore solitary species of plants cheat less often as compared to gregarious ones. Although the flower–pollinator mutualism is known for a long time, the presence of cheaters and the complexities in the mutualistic relationship came to light very recently. This is quite representative. A layman’s perception of evolution often does not go beyond what he feels Darwin must have said. In popular perception Darwin’s was the last word in evolution, and he established evolutionary theory once for all. The reality is far from it. Although what Darwin said was a major quantum leap in biological thinking, today’s evolutionary biology has progressed far beyond in terms of understanding the subtleties of the evolutionary process. The punch line of Darwinism—“survival of the fittest”—still remains grossly true, but we now understand that it is not as naïve as it looks. The cheater flower example is appropriate for demonstrating a number of important principles in evolution. There may not be a unique “good” character for a species as a whole. What is “good” for an individual and therefore what is selected by natural selection is highly contextual. For example if we assume that pollinators are good at learning, the optimum proportion of flowers a given plant should produce would depend upon what other plants are producing. If others are producing very few flowers with nectar, it makes sense for you to make more but just more enough for the pollinators to understand that you are more rewarding than others. By doing this you can attract more pollinators and increase your reproductive success as compared to others. But over several generations, this would lead to all plants making copious nectar and investing a lot in it. Somewhere along this axis, tables can turn once again. Since most plants are making copious nectar, pollinators need not worry much about learning and avoiding cheaters, and if this happens, a single or few cheaters can take advantage of it. This means that there may not be an all-time optimum strategy, and the optimum itself might undergo self-driven oscillations. This is quite contrary to a popular perception that evolution leads to “perfection.” The definition of “perfect” is itself not universally stable, and the short-term “goal” to be achieved by evolution may itself keep on changing. Alternatively there can be more than one point of “perfection,” and different genotypes in a single population may achieve different perfections. This is a very important concept for the arguments in this book, and we will elaborate on it once again later in this chapter when we learn about the theory of games. But before going there, we need to make our ideas clear about a few other issues.
Although the “optimum” is complex and contextual, individuals do not have to understand the complex context and make a conscious choice of the best option. Plants do not have a brain and therefore presumably do not understand what cheating is. They also cannot calculate the cost or benefit of a behavioral strategy, and yet the optimum behavioral strategy evolves. In this and several other examples, mathematical ecologists have calculated the optimum for a given situation, and the observed behavior of a species in the wild is very close to the calculated optimum. This apparent intelligence is built into the system by the process of evolution. So, even unintelligent organisms appear to have very wise strategies to face a variety of environmental situations and challenges, acquired through natural selection over generations.
There is no free lunch in evolution. The cheetah, for example, has evolved to be the fastest among ground mammals. But this does not come as a free lunch. There are costs associated with the running ability in terms of physiological and anatomical adjustments of the body needed to achieve the fastest speed. For aerodynamic reasons cheetahs need to have a small head. A large head like a lion will offer greater aerodynamic resistance. A smaller head necessitates a compromise in the power of the jaw muscles. Also as their survival depends on speed, cheetahs cannot afford to get injured. As a result, cheetahs are generally the losers if there is a conflict with other carnivore species on a kill. Similar to the cheetah, any organism has to bear some cost for achieving anything. In other words, if you want to gain something, it is often inevitable that you lose something else. Evolutionary biologists call it an evolutionary “trade-off.” The cheetah’s running speed is itself a good example of an evolutionary trade-off. There are umpteen examples of it in biology. Whether there are any achievements by any species in the history of evolution without involving any direct or indirect cost or a trade-off is doubtful.
Adaptations can take funny turns at times. Take the ability to type on a computer. When did we evolve this ability? Computers are so recent that not more than two generations have used them. Add the history of typewriters and a few more generations get added. But it takes several generations for any novel character to evolve. It is obvious that we did not evolve the ability to type on a keyboard. Our hands evolved to climb trees and then to make and use stone tools. But the hand structure evolved for making and using tools could be easily trained to type on a keyboard without any heritable biological change being involved. There is no doubt that some training and programming of neural circuits are necessary to be efficient in typing but no heritable change is involved. When an organ evolved because of one kind of selective pressure suddenly finds a new application and starts a new function it did not evolve for, the phenomenon is called exaptation [16]. Even an exaptation may have an associated trade-off. A hand making stone tools needs to have power and impact resistance. A skilled typing hand needs finer coordinated movements but hardly any power. If you keep on using your hands for typing alone for a long time, you will improve both speed and perfection in typing. At the same time, you are most likely to lose power and impact resistance, and if at all you have to make stone tools, you may find your hands entirely useless for this work. The modern human life is full of such exaptive changes, and we could be bearing quite some cost for it.
There is another limitation of the adaptive evolution paradigm that we need to understand well in order to understand the evolution of diabetes and related disorders. An adaptive character evolves by selective pressure faced by the population over a long time, in a way organisms evolve for their past, not for the present or the future. If there is a range of variation of an environmental parameter, organisms evolve to cope with that range, but they may fail to face the challenge if it goes beyond the range that it has evolved for. For example, every species has a range of temperatures that it can cope with. Some bacteria evolved in hot sulfur spring can grow at much higher temperatures demonstrating that life can exist at that temperature, but organisms not evolved for this range will not be able to tolerate that temperature. This meaning of an “out-of-range” stimulus is easy to understand. Situations can be funny if the environmental stimulus is out of range for what the species has evolved for but is not lethal.
Niko Tinbergen, one of the founders of ethology, the science of animal behavior, did an intriguing experiment several decades ago. While trying to answer the question “What features of an egg make a female gull recognize and incubate it?” Tinbergen made several versions of artificial eggs making serial changes in their shape, size, color, and markings. An interesting finding of these experiments was that females preferred to incubate large-sized eggs if the marking on them were similar to a real egg. This preference could be stretched to ridiculous levels. When offered a football-sized artificial egg, a female would typically try to sit on it ignoring her own real eggs. The intriguing observation was that although it was impossible to sit on the huge “egg,” she preferred the supernormal “egg” over the real eggs [17] (Fig. 1.1).
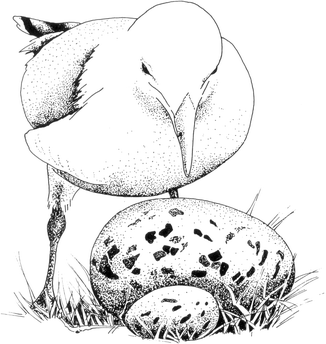
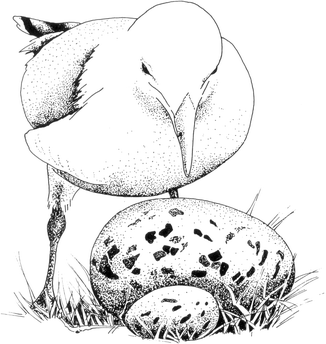
Fig. 1.1
Response to a supernormal stimulus: A gull female prefers an unrealistically large artificial “egg,” while she ignores her true eggs (Redrawn from Tinbergen) [19]
Stimuli such as a football-sized egg are called supernormal stimuli. Why does the mother gull give an exaggerated response to the supernormal stimulus is not very difficult to understand. Naturally there will be some variation in egg sizes. It has been shown in several species of birds that the parents preferentially feed the larger and stronger of the chicks. One with a wider gape and stronger begging behavior gets a larger share in food and parental care. This is adaptive since this way the parents ensure that their next generation is stronger and more competitive. Therefore, naturally, birds show a slightly higher preference for larger offspring while tendering or feeding. The same could be true for incubating eggs. Among the natural size variants, the mother gull could be showing slight preference for the larger ones. So the tendency that could have evolved is to prefer the larger egg. The question of how large a real egg could be did not arise during evolution since the natural size range was very narrow, and before the crazy ethologist, nobody gave them a football-sized egg. When the experimenters offered that, it worked as a supernormal stimulus and evoked a response directly proportional to its size. This is an example of how a supernormal stimulus can evolve a maladaptive response.
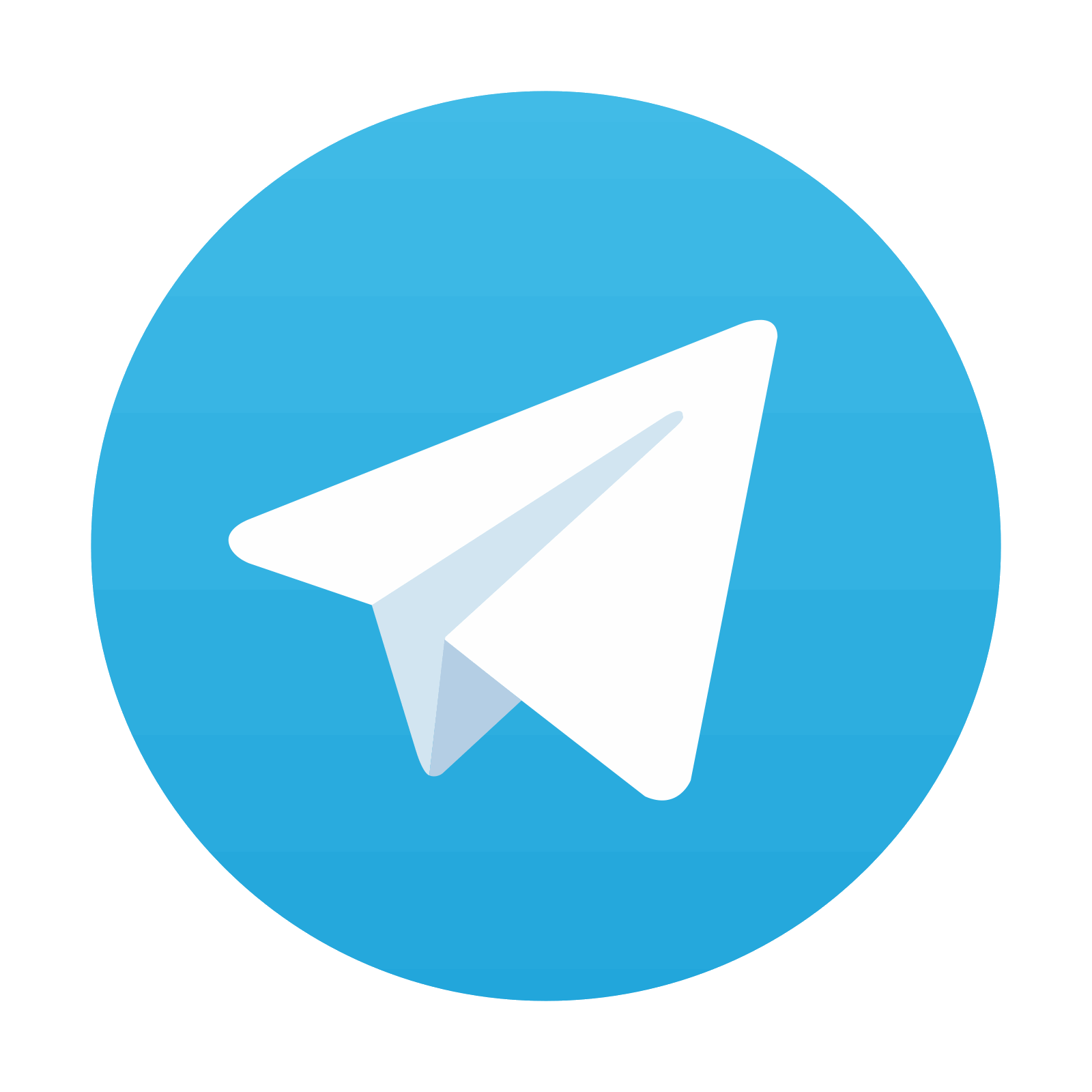
Stay updated, free articles. Join our Telegram channel
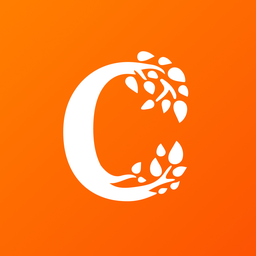
Full access? Get Clinical Tree
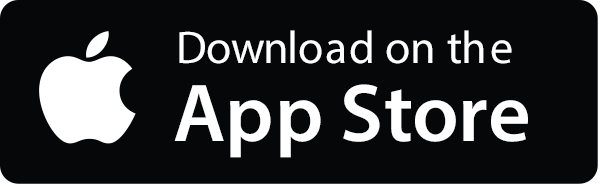
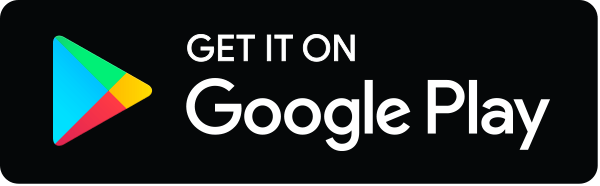