CHAPTER 7 Ryan Wheeler1 and Jan Kovacic1,2 1 Four Seasons Animal Hospital, Lafayette, California 2 Horizon Veterinary Services, Lafayette, California The acid–base status of critically ill patients is a complex interplay of multiple dysfunctional organs, depleted buffer systems, mixed acid–base disorders, and conflicting homeostatic mechanisms. It is often complicated by iatrogenic problems associated with imprecise fluid therapy, unforeseen effects from medications, and unanticipated complications when the treatment for one acid–base disturbance ends up creating another. Every acute, life‐threatening illness or injury will eventually cause changes in acid–base status, sometimes within minutes of the inciting cause. Every chronic illness that becomes life threatening will eventually change acid–base balance. Therefore, acid–base monitoring should be viewed as a window into serious illness. Finding alterations in acid–base status provides an alert that the patient and their problems may be more serious than anticipated. The goal is to unmask the components of the acid–base disturbance as early as possible and specifically treat the underlying cause. Acidosis is the term for acidifying events and alkalosis is the term for alkalinizing events. The terms acidemia and alkalemia have been used to describe the acid or alkali status of the blood. However, these terms, as previously defined, are not entirely accurate nor descriptive of the true acid–base status of the blood. A patient with a blood pH <7.4 has been termed “acidemic.” However, the neutral pH of an aqueous solution at the body temperature of 37 °C is 6.8, so the pH of blood is almost never on the acid side of neutral. At best, the term acidemia should be used for an increase in blood acidity and alkalemia for a decrease in blood acidity [1–3]. Terms commonly used when discussing acid–base status are provided in Box 7.1. There are multiple homeostatic mechanisms, chemical and physiological, intra‐ and extracellular, that control the hydrogen ion concentration ([H+]) of body fluid compartments. A normal [H+] of 40 nmol/L is reported as pH = 7.4, and the range compatible with life is 15–158 nmol/L (pH = 6.8–7.8). Acidity increases with an increase in [H+], but the pH value goes down. Buffer systems of weak acids and their conjugate bases help to control hydrogen activity through their relative affinity for protons. Peripheral chemoreceptors in the aortic and carotid bodies sense [H+] and trigger renal and pulmonary mechanisms to normalize pH. Central chemoreceptors in the medullary respiratory center of the brain also sense changes in the partial pressure of carbon dioxide in plasma (PCO2) independent of changes in pH, a critical step in the rapid ventilatory response to metabolic changes that alter [H+]. The kidney is equipped to respond to changes in the plasma [H+], bicarbonate (HCO3−), sodium (Na+), potassium (K+), chloride (Cl−), CO2, angiotensin, and aldosterone, all of which affect [H+]. The liver is a net producer or consumer of H+ depending on diet, metabolism, volume status, perfusion, medications, toxins, and fluids. Even the skeletal system plays a role in acid–base balance by contributing calcium carbonate as a buffer [4]. More important is the H+ environment in which most of the body’s work is done – the intracellular space. Intracellular pH is close to neutral (6.8–7.1) and can be acidic (pH <6.8) without serious deleterious effects on the cells. Intracellular pH is also more tightly controlled than extracellular pH. For example, lymphocytes are able to maintain an almost constant intracellular pH = 7.1 + 0.06 when exposed to a blood pH = 6.8–7.4 [5]. The cells have some defense against extracellular pH changes, making cellular metabolism relatively resistant to changes in blood pH. Unfortunately, there is currently no technology available in the clinical setting to measure intracellular pH. The critical importance of acid–base balance lies in the fact that extremes in [H+] can be reached within minutes due to cardiopulmonary arrest, shock, trauma, hypo‐ or hyperventilation, or toxins, and within hours due to almost any serious medical condition. The pH and [H+] reported as lethal are listed in Table 7.1. Extreme changes in [H+] cause loss of function of membrane channels, especially in critical cardiac, brain, and nerve cells, alter the structural and functional integrity of proteins causing changes in enzyme function, and disrupt K+ balance between intra‐ and extracellular fluid [3,6,7]. In addition, patient therapy can play a role in creating critical acid–base disturbances, usually due to the unanticipated deleterious effects of drug and fluid therapy. For this reason, when treating critical patients, acid–base monitoring is essential. Table 7.1 Range of pH and H+ compatible with life. The mantra of critical care medicine is “treat the underlying cause.” Never is this more important than in the clinical application of acid–base physiology. Rarely is an acidemia or alkalemia so severe that the objective is to treat the disturbance without regard to etiology. The real value in acid–base analysis is to discover the multiple compensating and counterbalancing causes that are common in critical patient care. Therefore, the goal of therapy is not to treat the acidemia or alkalemia per se. Physiological compensation or the counter‐balancing effects of mixed disorders (acidosis + alkalosis) may even make a serious disorder appear less severe than it really is. It is important to uncover and treat the underlying causes which may be extreme even if the acidemia or alkalemia is not. Fortunately, we have many tools to use in this process. The primary goal is to identify and quantify the effect made by each agent known to cause changes in acid–base balance, within the limits of our current understanding and of available analyzer technology. The two most popular descriptions of acid–base physiology are the Henderson–Hasselbalch (HH) approach, also termed the “traditional” approach, and the Stewart strong ion approach, also termed “nontraditional.” Common biochemical formulas used to define each method are provided in Box 7.2 and Table 7.2. The HH approach provides a simple means for identifying respiratory or metabolic acidosis or alkalosis, and whether appropriate compensatory mechanisms are present (Box 7.3 and Table 7.3); however, this method is incomplete in evaluation of independent variables. The biggest problem with each of these approaches is that, except for PCO2, the endpoints of their analysis reflect a summation of multiple physiological or pathological processes without isolating or quantifying the effect of any individual causative agent. Many of the major concerns and inadequacies of the traditional and nontraditional methods of acid–base assessment are outlined in Table 7.2. Table 7.2 Comparison and Clinical Limitations of Traditional (HH) and Non‐Traditional (Stewart) Acid‐Base Analysis. Table 7.3 Expected compensation for metabolic and respiratory acidosis and alkalosis with intact physiological mechanisms. Source: adapted from DiBartola S. Introduction to acid–base disorders. In: Fluid, Electrolyte, and Acid–base Disorders in Small Animal Practice, 4th edn. St Louis: Saunders, 2012: pp 231–52. Acid–base analysis became clinically useful when researchers expanded on HH and Stewart acid–base physiology to describe a semiquantitative approach that yields the approximate contribution of measurable agents (independent variables) known to cause acid–base disturbances [8–11]. Through experimental evidence, formulas were derived that permit the calculation of the contribution that each agent makes to metabolic acid–base status as reflected in base excess (BE). These formulas vary from species to species. The calculations are inherently approximations, hence the term “semiquantitative.” This approach allows the clinician to (1) identify each contributing factor, (2) isolate cause effect, (3) target interventions on normalizing each contributing factor, (4) support the homeostatic mechanisms that will help to normalize each factor, and (5) avoid therapies that can interfere with normalization or create acid–base disturbances on their own. The sum of the effects of the measured agents, if different from the total BE, reveals that the difference is due to agents yet unidentified and unmeasured. This directs supplemental testing that can help identify, if not quantify, the unidentified factors altering acid–base balance. In veterinary medicine, there is evidence that this semiquantitative acid–base (SQAB) approach is more clinically useful than either the HH or Stewart methodology [12–14]. The agents that create change in acid–base status all play critical roles in cellular function – membrane structure, membrane integrity, membrane potentials, enzyme structure, enzyme kinetics, electron transport, proton motive force, channels, transporters, energy metabolism, hormone interactions, second messengers, cell signaling, neuromuscular function, neuron function, and the like. Disruptions in these functions often become life threatening even before they create a significant acidemia or alkalemia, and even when acidemia or alkalemia is not significant because of counterbalancing disturbances or therapies. This is the primary reason why acid–base disorders are treated early and why therapy is directed at the underlying cause. Important contributions to the underlying factors contributing to acid–base disorders can be derived from the initial history, physical examination, minimum database, and clinicopathological testing. A specific SQAB profile can be created to expedite analysis of the acid–base status of the patient (Box 7.4). Diagnostic imaging can be necessary to further define the causal disorder. Signalment (age, sex, breed), past medical history, drug therapy, noted alterations in drinking, urination, defecation, vomiting, weight loss, respiratory changes, behavior and mentation, and possible toxic exposures are all important when collecting a history. Historical information may provide insight into the nature of the acid–base disturbance. A list of physical examination findings associated with acidemia and alkalemia is provided in Table 7.4 and covers the entire spectrum of serious illness. It is important to remember that this association does not imply that the acidemia or alkalemia is the direct biochemical cause of these signs. Continuous monitoring of physical parameters such as temperature, pulse rate, pulse quality, heart rate and rhythm, respiratory rate, respiratory effort, mentation, mucous membrane color, and capillary refill time is essential for early detection of problems resulting from or causing changes in acid–base balance. Table 7.4 Clinical signs associated with acidosis and alkalosis. ECG, electrocardiogram; GI, gastrointestinal. The minimum database consists of packed cell volume (PCV), total proteins (TP), blood glucose, blood urea nitrogen (BUN), electrolytes, and blood gas analysis. The PCV is important in determining the contribution that hemoglobin makes to the assessment of pH. The remainder of the database will contribute to the SQAB profile (see Box 7.4). A complete blood count, serum biochemistry, and urinalysis provide important data for identifying factors potentially contributing to acid–base disorders. There are no analyzers that will assay all of the necessary SQAB analytes from one sample. Therefore, an important step for SQAB analysis is to equip the ICU with the point of care (POC) analyzers (blood gas, electrolyte, biochemistry) that can provide SQAB profile results rapidly and efficiently. Point of care analyzers should be selected that use minimal sample size and provide rapid sample processing. It is important to use a brand of analyzer that has been validated in animals and for which reference ranges for the species being studied have been provided. Data is only useful if it is free from errors in sampling or laboratory technique, and if it is obtained often enough to reflect important changes in a patient’s condition. The frequency of testing intervals should be based on the severity of illness, the presence of unstable trends in monitored parameters, and the presence of known or anticipated etiologies that cause rapid changes in acid–base status. It is important to: (1) draw blood samples only in the frequency and quantity needed, to prevent significant patient blood loss, (2) use lyophilized or minimal volume of anticoagulant to prevent dilution of the sample, and (3) run the tests with minimal delay from time of sample collection. Diagnostic imaging will typically include plain thoracic and abdominal radiographs. Assessment of the lungs and pulmonary vasculature can aid in the assessment of respiratory acid–base changes. Should the lungs appear normal, changes in PCO2 may reflect physiological compensation for metabolic acid–base disturbances. Normal lungs also suggest that the patient will probably be able to expire any additional CO2 that is produced (such as after bicarbonate administration). Imaging of the heart may support the presence of heart disease, which could be a contributor to the acid–base disturbance or to a lack of response to therapy. A small vena cava and cardiac silhouette may indicate a hypovolemic state. Ultrasound is used in evaluation of the heart, vessels, gastrointestinal tract, pancreas, spleen, liver, kidneys, and bladder. Abnormalities could support an underlying cause or demonstrate a serious consequence of an acid–base disorder. In seriously ill patients, change in acid–base balance can be continuous and dynamic. The life‐threatening potential of the causes and effects of acid–base disorders necessitates POC testing and intensive monitoring. Monitoring intensity can be decreased when: (1) response to therapy is predictable and appropriate, (2) repeated results stabilize into favorable trends, and (3) the patient’s overall condition (not just acid–base status) is improving. Blood pressure is monitored to detect alterations in cardiac output and peripheral vascular resistance, each potentially a cause or result of acid–base disturbances (see Chapter 3). End‐tidal CO2 monitoring (ETCO2) provides a continuous reflection of the PCO2 in the blood (see Chapter 8). This can provide a direct indication of respiratory acidosis or alkalosis, whether it is physiological (compensatory) or pathological. During early recovery from cardiopulmonary arrest, a rise in ETCO2 helps to confirm return to spontaneous circulation, restoration of oxidative metabolism, and clearance of acidity in the tissues as CO2 is transported to the lungs for excretion. Pulse oximetry (see Chapter 8) measures the oxygen saturation of hemoglobin (SpO2), a major component of oxygen delivery (DO2) and of the maintenance of oxidative metabolism. A significant decrease in SpO2 and DO2 can lead directly to anaerobic metabolism and severe lactic acidosis. It can also lead indirectly to a full spectrum of acid–base changes due to complete disruption of cellular physiology. Continuous electrocardiographic monitoring (see Chapter 11) can demonstrate changes in heart rate, rhythm, and electrical activity, which could cause acid–base abnormalities or result from changes in acid–base status. If a dysrhythmia disrupts cardiac output, the alteration in DO2 can create global problems down to the cellular level. The ability to continuously monitor many of the parameters of acid–base analysis (PO2, PCO2, glucose, electrolytes, lactate) is under development using subcutaneous probes that measure these analytes in interstitial fluid or intravascular probes that analyze blood. The technology is costly and of limited use at this time since many of the important parameters needed for complete acid–base analysis are not reported. Currently, the most useful continuous monitoring is ETCO2 during anesthesia, ventilator therapy, or during treatment and recovery of cardiopulmonary arrest. The use of SQAB analysis offers a better understanding of the factors contributing to acid–base disturbances. Because the sickest patients often have multiple disturbances, the therapeutic response can be more precise and less likely to create or exacerbate other problems. With this improved understanding, acidemia or alkalemia per se is rarely treated; instead, treatment is directed at the underlying cause(s). Currently, cautious attempts to treat acidemia or alkalemia directly are reserved for when efforts to treat the underlying causes have failed. Contributions from Na+, K+, Cl−, phosphate, proteins (albumin), and lactate are recognized through the SQAB analysis, with each becoming a potential therapeutic target. When a new therapy has been initiated, frequent monitoring is necessary to insure that the desired and predicted effect is confirmed. When a response to therapy is not as predicted, a search for additional causes ensues. Often, homeostatic mechanisms have priority over acid–base balance, such as the maintenance of effective circulating volume. As in all systems of acid–base analysis, pH is the final summation of all acid–base disturbances, metabolic and respiratory. Base excess and [HCO3−] are parameters that sum the effects of all metabolic disturbances. Using the SQAB method, the standard BE is compared with the sum of the individual metabolic effects to unmask acid–base effects from unmeasured anions or cations. Since it is not a causative agent of acid–base change, [HCO3−] is of little importance. It is included to compare the SQAB profile to HH analysis. The plasma [H+] in dogs and cats is approximately 40 nmol/L, with cats normally slightly more acidic than dogs. In comparison, K+ is 100 000 times more concentrated than H+ in plasma; Na+ and Cl− are 3 000 000 times more concentrated. One of the variables that alters hydrogen ion activity, and therefore pH, is temperature. Aqueous solutions are neutral when hydrogen ion equals hydroxyl ion, H+ = OH−. This occurs at pH = 7.0 when the temperature of the solution is 25 °C. However, at body temperature (37 °C), neutral pH = 6.8. Hydrogen ion activity increases with increased temperature and the pH is lower [1,15]. The pH range compatible with life (6.8–7.8; see Table 7.1) represents a 10‐fold difference in H+, a wider range than is tolerated with other important ionic species. However, because normal [H+] is so small (40 nmol/L), this range represents a change in H+ of only 0.000143 mEq/L. It is remarkable that the body can sense and adjust to such minute changes in H+ and that clinicians can successfully intervene, without doing more harm than good, when homeostatic mechanisms are overwhelmed by illness or injury. There is accumulating evidence that increased extracellular H+ may be well tolerated and may even have a protective effect in some clinical situations. Permissive hypercapnia in the management of acute respiratory distress syndrome (ARDS) is a ventilator strategy that is considered to be beneficial to the patient when compared to the damage to the lung that may occur with greater tidal volumes and inspiratory pressures [16]. Acidemia may improve outcomes in ARDS patients and there is evidence that buffering respiratory acidosis may exacerbate the condition. Animal studies have demonstrated that acidemia can protect myocardial and hepatic cells during hypoxia [17]. A lower pH increases the release of oxygen from hemoglobin, thereby increasing oxygen extraction by the tissues. In exercising athletes (with lactic acidosis and pH as low as 7.0), this mechanism can account for up to 60% of the oxygen available to the tissues. Experimental levels of arterial PCO2 up to 1 atmosphere (750 mmHg) and intracellular brain pH of 6.2 have been tolerated in rats for 15 minutes without neurological impairment [17–19]. Acidemia has been found to attenuate inflammatory processes, including leukocyte superoxide formation, apoptosis of nerve cells, phospholipase A2 activity, expression of cell adhesion complexes, and xanthine oxidase activity [16,20,21]. Studies have shown, however, that the body reacts differently depending on the source of substances creating the acidemia. Cardiac myocytes show a faster increase in intracellular [H+] caused by increased PCO2 compared to hydrochloric acid (HCl), a fixed acid. This increased acidity does decrease myocardial contractility, but it also decreases myocardial oxygen consumption that may have a short‐term protective effect. When brain acidosis is a result of CO2 rather than HCl, there is less free radical production and less lipid peroxidation. Acidosis caused by CO2, compared to acidosis from HCl, has an inhibitory effect on lactate production in the tissues [16,18,20,21]. At this early stage of our understanding of alkalemia and acidemia, the take‐home message is that alkalemia may be far more harmful than a comparable acidemia, and that a change in H+ activity is almost always less important than the underlying cause of the change. An increase in PCO2 >44 mmHg (hypercapnia) is also termed hypoventilation and creates a respiratory acidosis, with low pH, high H+, and a compensatory increase in HCO3−. The development of respiratory acidosis can be classified as acute or chronic, which will subsequently influence the degree of metabolic compensation that has been demonstrated in dogs and cats (see Table 7.3) [22]. Respiratory acidosis may result from any disease process that can affect neuromuscular control of ventilation, loss of small or large airway integrity, or alveolar gas exchange. Common causes include large airway obstruction, respiratory center depression, increased CO2 production, impaired alveolar ventilation, restrictive extrapulmonary disorders, parenchymal and small airway diseases, improper mechanical ventilation, and obesity. Clinical signs of respiratory acidosis may be more indicative of the underlying disease process than of the hypercapnia itself. These are typically more severe in patients with acute disorders (such as congestive heart failure, pneumonia) than in those with chronic, compensated respiratory disorders. Hypercapnia can also trigger cardiovascular changes such as tachycardia and vasodilation. Vasodilation can be of clinical significance in patients with intracranial disease; the increase in intracranial volume can cause a dramatic increase in intracranial pressure. Altered mentation is known to occur when PCO2 is significantly elevated. Treatment of respiratory acidosis is aimed at the underlying cause. Oxygen therapy is indicated in the treatment of hypoxemia caused by hypoventilation. In cases of airway obstruction, sedation, oxygen therapy, and airway management may be necessary to allow adequate ventilation. In some instances, mechanical ventilation may be required until the underlying disease process is corrected. Treatment with sodium bicarbonate or other alkalizing agents should be undertaken with extreme caution because, without mechanical ventilation, the patient may not be able to remove the CO2 that is produced when these agents are metabolized [3,23–25]. A decrease in PCO2 <36 mmHg (hypocapnia) is also termed hyperventilation and creates a respiratory alkalosis, with high pH, low H+ and a compensatory decrease in HCO3−. The development of respiratory alkalosis can be classified as acute or chronic, which will subsequently influence the degree of metabolic compensation that may be expected (see Table 7.3) [24]. Respiratory alkalosis may result from any disease process that can increase alveolar ventilation above what is necessary to expire the CO2 produced through normal metabolic processes. Common causes include hypoxemia, pulmonary parenchymal disease independent of hypoxemia, centrally mediated hyperventilation, overzealous mechanical ventilation, or situations that induce pain, fear, or anxiety. Treatment is aimed at addressing the underlying cause, and may include sedation, pain medications, and diuretics. Oxygen should be provided when hypoxemia contributes to respiratory alkalosis [24–26]. Sodium is the major extracellular cation and exists in the ionic form in body fluids at any pH. The contribution that Na+ makes to the SQAB analysis is expressed in the formulas shown in Box 7.5.
Acid–base status
Introduction
pH
[H+] nmol/L
[H+] mmol/L
[H+] mEq/L
Lethal
6.8
158
0.000158
0.000158
Normal
7.4
40
0.000040
0.000040
Lethal
7.8
15
0.000015
0.000015
Methods of acid–base analysis
Parameter
Traditional
Non‐Traditional
Notes
Terms used
Acid‐Base diagnoses
pH
plays a role in determining pH
) based on its dissociation constant (pKa).
Base Excess (BE)
Anion Gap (AG)
Strong Ion Difference (SID)
SID=[Na+]+[K+]+[Ca++]+[Mg++] − [Cl] − [lactate] − [ketoacids]
ATOT
pCO2 and Compensation
Metabolic acidosis
↓
HCO3− of 1 mEq/L results in a
↓ in PCO2 of 0.70 mmHg
Metabolic alkalosis
↑
HCO3− of 1 mEq/L results in an
↑ in PCO2 of 0.70 mmHg
Respiratory acidosis– acute
↑
PCO2 of 1 mmHg results in an
↑ in HCO3− of 0.15 mEq/L
– chronic
↑
PCO2 of 1 mmHg results in an
↑ in HCO3− of 0.35 mEq/L
Respiratory alkalosis– acute
↓
PCO2 of 1 mmHg results in a
↓ in HCO3− of 0.25 mEq/L
– chronic
↓
PCO2 of 1 mmHg results in a
↓ in HCO3− of 0.55 mEq/L
Initial diagnostics and monitoring
History and physical examination
Clinical signs seen with acidosis
Clinical signs see with alkalosis
Respiratory
Hyperventilation
Brady‐ or tachypnea
Cardiovascular
Poor perfusion
Abnormal blood pressure
Brady‐ or tachycardia
Decreased cardiac contractility
ECG ST changes
Dysrhythmias
Coronary vasodilation
Cerebral vasodilation
Systemic vasodilation
Neurological
Altered mentation
Cognitive dysfunction
Blunted cranial nerve reflexes
Abnormal musculoskeletal reflexes
Stupor, coma, death
Hemolymphatic
Altered O2‐hemoglobin affinity
Coagulation disorders
Genitourinary
Anuria, oliguria, polyuria
Gastrointestinal
Anorexia
Vomiting
Diarrhea
Altered GI motility
Musculoskeletal
Muscle weakness
Myoclonus
Endocrine/metabolic
Protein alterations
Hormone dysfunction
Enzyme dysfunction
Respiratory
Hypoventilation
Brady‐ or tachypnea
Cardiovascular
Poor perfusion
Abnormal blood pressure
Brady‐ or tachycardia
Altered cardiac contractility
ECG ST changes
Dysrhythmias
Coronary vasodilation
Cerebral vasoconstriction
Systemic vasoconstriction or dilation
Neurological
Altered mentation
Cognitive dysfunction
Blunted cranial nerve reflexes
Abnormal musculoskeletal reflexes
Stupor, coma, death
Hemolymphatic
Altered O2‐hemoglobin affinity
Coagulation disorders
Genitourinary
Anuria, oliguria, polyuria
Gastrointestinal
Anorexia
Vomiting
Diarrhea
Altered GI motility
Musculoskeletal
Muscle weakness
Myoclonus
Endocrine/metabolic
Protein alterations
Hormone dysfunction
Enzyme dysfunction
Point of care testing
Diagnostic imaging
Equipment‐based monitoring
Disorders of acid–base balance
Disorders of pH, HCO3−, and BE
Disorders of CO2
Disorders of Na+ or free water
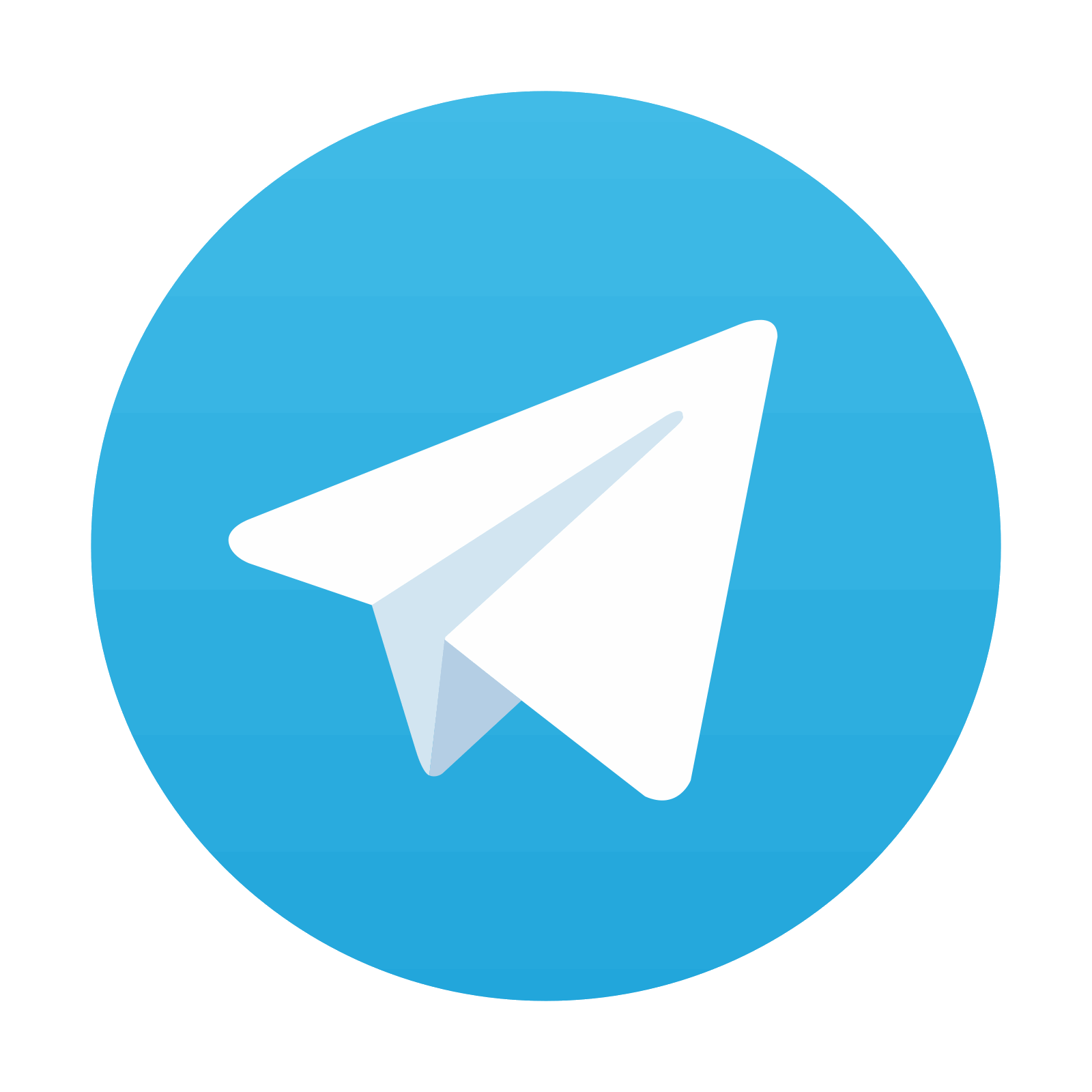
Stay updated, free articles. Join our Telegram channel
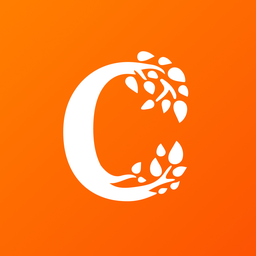
Full access? Get Clinical Tree
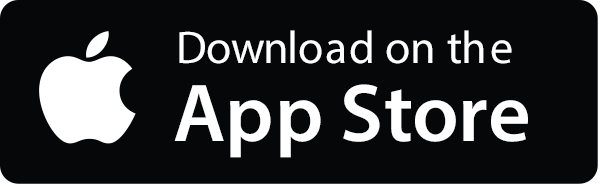
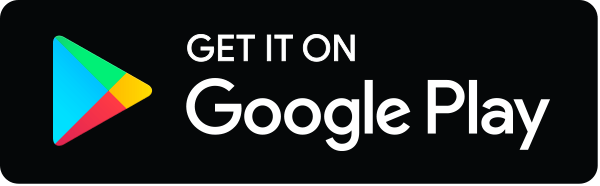