CHAPTER 6 Linda Barton1 and Rebecca Kirby2 1 BluePearl Specialty and Emergency Medicine for Pets, Renton, Washington 2 (Formerly) Animal Emergency Center, Gainesville, Florida Electrolytes are substances that dissociate into charged particles (ions) in solution and acquire the capacity to conduct electricity. It is the gain or loss of electrons that creates a negative or positive charge, respectively. Positive ions, known as cations, often attract negative ions, known as anions, and form an ionic bond. These bonds can be found in many biological compounds such as acids, bases, and salts. However, these ionic bonds will break when added to water. Sodium (Na), potassium (K), chloride (Cl), calcium (Ca), magnesium (Mg), and phosphorus (P) are the electrolytes that play a critical role in maintaining normal cellular functions. These ions will regulate or affect two important physiological functions: the flow of water molecules and the electrical charge across the cell membrane. Electrolytes are constantly lost from the body through the urine and gastrointestinal secretions. Therefore, the regulation and preservation of normal concentrations of these important electrolytes can be crucial for supporting life‐sustaining functions, such as cardiac performance, vascular tone, brain function, neuromuscular activity, and fluid balance. Electrolytes (ions) cannot diffuse passively across cell membranes. Their concentrations are therefore regulated by facilitated diffusion and active transport. Facilitated diffusion occurs through protein‐based channels, which allow passage of the solute along a concentration gradient. In active transport, energy from adenosine triphosphate (ATP) changes the shape of membrane proteins that move ions against a concentration gradient [1]. The flow of water molecules across cell membranes is primarily dependent upon the concentration of Na, and to a lesser extent the K concentration. The extracellular fluid (ECF) compartment volume is maintained by Na salts and the intracellular fluid (ICF) compartment by K salts. Because the cell membrane is freely permeable to water but not to ions, the ECF and ICF spaces will strive to establish an osmotic equilibrium. Water will flow from the compartment of lower osmolarity to the compartment with a higher osmolarity until the osmotic pressures are equalized across the cell membrane. This state is not a true equilibrium but rather a steady state achieved by active transport by the Na/K pump and other interrelated channels and transporters [1]. An increase in osmolarity of the ECF can result in cellular dehydration and conversely a decrease in ECF osmolarity can result in cellular overhydration. The electrical charge across the cell membrane is a function of Na, K, Cl, Ca, and Mg. The action potential or nerve impulse is a transient alteration of the transmembrane voltage across an excitable membrane in an excitable cell (such as a neuron or myocyte). This is generated by the activity of voltage‐gated ion channels embedded in the cell membrane. An action potential develops when the threshold of the cell membrane potential is reached. Pulse‐like waves of voltage travel along the axons of neurons and myocytes (Figure 6.1) and result in electrical activity such as nerve impulses, muscle contraction, and cardiac conduction. Figure 6.1 Pulse‐like waves across the cell membrane of the cardiac myocyte are a result of shifting of electrolytes and initiate the action potential. Phase 0 causes depolarization. Rapid sodium (Na) channels are stimulated to open, flooding the cell with positive Na ions. This causes a positively directed change in the transmembrane potential. Depolarization of one cell triggers the Na channels in surrounding cells to open as well, causing the depolarization wave front to propagate cell by cell throughout the heart. Phase 1 is the initial stage of repolarization. The voltage is rapidly lowered by the movement of chloride (Cl−) ions into the cell. Phase 2 (the plateau stage) is where the rate of repolarization is slowed by the influx of calcium (Ca) ions into the cell. The Ca ions enter the cell more slowly than the Na ions and help prevent the cell from repolarizing too quickly, thus extending the refractory period. This mechanism helps regulate the rate at which cardiac tissue can depolarize. Phase 3 is the later stage of repolarization where intracellular potassium (K) leaves the cell in an effort to restore the resting membrane potential (−90 millivolts (mv)). Once repolarization is complete, the cell will be able to respond to a new stimulus. Phase 4 occurs after repolarization is complete and restores the Na and K balance to the original intracellular and extracellular concentrations. Each major cation (Na, K, Ca, Mg) and anion (Cl, P) has an individual and specific role to play in the function and homeostasis of every cell in the body. The charge, location, and concentration of each electrolyte contribute to the role it plays in a cell and can affect the function and concentration of other electrolytes. Sodium is the major cation in ECF and thus the major contributor to extracellular (including plasma) osmolarity. The plasma osmolarity is maintained within narrow limits by balancing water intake with water losses. Small elevations in the plasma osmolarity are sensed by osmoreceptors in the hypothalamus. Thirst and water excretion are both altered in response to the secretion of antidiuretic hormone (ADH, also called arginine vasopressin). The secretion of ADH occurs in response to increased plasma osmolarity or decreased effective circulating volume. The ADH binds with vasopressin (V2) receptors in the distal tubules and collecting ducts of the kidneys, stimulating the activation of renal epithelial Na channels. The resultant Na resorption contributes to the corticomedullary osmotic gradient that is necessary for maximum water absorption. This allows free water resorption, hence lowering sodium and increasing vascular volume [2]. In addition to the role that Na plays in fluid balance, the transmission of Na into and out of the cell is a critical part of many cellular functions. The transmembrane movement of Na is particularly important in the generation of electrical signals or currents in the brain, spinal cord, peripheral nervous system, myocardium, and muscles. Potassium is the major intracellular cation, playing an important role in neuromuscular transmission as well as other vital cell functions. The resting cell membrane potential is dependent upon a higher intracellular to extracellular ratio of K. The K concentration must be tightly regulated. Both insulin and beta‐adrenergic catecholamines will increase cellular K uptake by stimulating cell membrane Na/K–ATPase. The kidneys are primarily responsible for maintaining the total body K, matching excretion with intake. Under most homeostatic conditions, K delivery to the distal nephron remains small and is fairly constant. By contrast, the rate of K secretion by the distal nephron varies and is regulated according to physiological needs. Aldosterone will upregulate the basolateral Na/K pump in the principal cells in the distal renal tubule, creating a concentration gradient for the resorption of Na and the secretion of K [3]. Aldosterone synthesis is stimulated by increased concentrations of K, as well as angiotensin II, adrenocorticotropic hormone (ACTH), acidosis, and atrial stretch receptors. Calcium, as calcium phosphate, acts as supporting material in bones, with 99% percent of the total body Ca residing in the bones and teeth. The remaining 1% of the Ca performs important life‐sustaining cellular functions. Calcium is involved in maintaining the stability of fibrin, the transmission of nerve impulses, the flow of fluid through cell membranes, and the contraction and relaxation of muscle. In addition, the Ca ion is one of the most widespread second messengers for cellular signal transduction, particularly those related to neuromuscular function and cardiac conduction. Endothelial Ca ions regulate the production of nitric oxide and the stimulation of K channels to efflux K, causing hyperpolarization and vascular smooth muscle relaxation [4]. The cytoplasmic concentration of Ca ions is a consequence of Ca ion passage through the cell membrane by way of Ca‐binding proteins or voltage‐gated calcium channels. Calcium ions can also be released into the cytoplasm from intracellular Ca stores, such as the endoplasmic or sarcoplasmic reticulum and mitochondria. However, excessive intracellular calcium may damage the cell or even cause it to undergo apoptosis, or death by necrosis. Therefore, the quantity of intracellular calcium must be tightly regulated. Extrusion of Ca from the cell is facilitated by transport proteins such as the Na/Ca exchanger and Ca/ATPase plasma exchanger. Ionized calcium is hormonally regulated and normally maintained within narrow limits by the actions of parathyroid hormone (PTH), calcitonin, and vitamin D. Low calcium levels trigger PTH release, which promotes bone mineral dissolution and increased renal reabsorption of Ca. PTH also increases renal hydroxylation of inactive vitamin D to calcitriol, which increases gastrointestinal absorption of dietary calcium. Increased serum calcium and calcitriol levels inhibit PTH secretion. Calcitonin is released from the thyroid gland in response to high Ca concentration. As opposed to PTH, calcitonin inhibits bone resorption of Ca and renal reabsorption of Ca and P. Magnesium is the second most abundant intracellular cation, with most of the total body Mg found in bone and skeletal muscle. In addition, Mg is a co‐factor to more than 300 enzyme‐driven biochemical reactions occurring in the body [5]. Magnesium influences the activity of enzymes by: Intracellular Mg will block Ca and K channels, regulating Ca and K entry into the cell [7,8]. By competing with Ca for membrane binding sites and by stimulating Ca sequestration by sarcoplasmic reticulum, Mg helps to maintain a low resting intracellular Ca ion concentration. Magnesium has a key role in many other important biological processes such as cellular energy metabolism, cell replication, and protein synthesis. The electrical properties of membranes and their permeability characteristics are also affected by magnesium. It affects myocardial contractility by influencing the intracellular Ca concentration and the electrical activity of myocardial cells. Magnesium affects the movement of ions such as Na, K, and Ca across the sarcolemmal membrane of the myocardial conduction system [9]. It may also affect the vascular smooth muscle tone. Intracellular Mg is maintained within narrow concentration limits except in extreme situations such as hypoxia or prolonged Mg depletion. Very little is known about the mechanisms involved in the regulation of intracellular Mg [10]. Most Mg is bound to anionic compounds such as ATP, ADP, citrate, proteins, RNA, and DNA or is sequestered within mitochondria and endoplasmic reticulum. The kidney plays a major role in Mg homeostasis and the maintenance of plasma Mg concentration. Most of the filtered Mg (65%) is resorbed in the ascending loop of Henle [10]. The plasma Mg concentration is a major determinant of urinary magnesium excretion. Several hormones including PTH, ADH, calcitonin, glucagon, and insulin have been shown to affect Mg reabsorption [11,12]. Chloride is the most abundant anion in the extracellular fluid and the second most important contributor to plasma tonicity. Chloride is distributed primarily to the ECF compartment and can diffuse easily across vascular endothelial membranes. Chloride transport is closely linked to Na movement. This anion helps to regulate osmotic pressure differences between fluid compartments, having an important role in maintaining proper hydration and balancing cations in the ECF to maintain electrical neutrality. Renewed interest in the physicochemical approach to acid–base analysis (Stewart approach and semiquantitative acid–base analysis) has refocused attention on Cl as a major determinant of acid–base status (see Chapter 7). The Cl shift within the blood helps to move bicarbonate ions out of the red blood cells and into the plasma for transport. In the gastric mucosa, chlorine and hydrogen combine to form hydrochloric acid. The kidney excretes chloride, where the paths of secretion and reabsorption of Cl ions follow the paths of Na ions. Aldosterone therefore plays an indirect role in Cl regulation. Phosphorus is the major intracellular anion, with most of the P located in the bone. Less than 1% of the total body P is located within the ECF and ICF spaces. The intracellular organic phosphate is an important constituent of nucleic acids, phospholipids, enzymatic phosphoproteins, and nucleotide co‐factors for enzymes and proteins. Cytosolic phosphate regulates intracellular reactions such as glucose transport, lactate production, and ATP synthesis [13]. Phosphorus also acts as both a urinary and a body buffer. Urinary phosphate constitutes the majority of titratable acidity. Phosphorus shifts into cells in response to alkalemia. Similar to Ca homeostasis, PTH, calcitonin, and vitamin D regulate phosphate intestinal absorption, deposition, and resorption from bone and renal excretion. In response to low serum phosphate concentration, calcitriol increases gastrointestinal P absorption and the kidneys decrease phosphate excretion. Elevated serum PTH levels promote rapid renal phosphate excretion. Electrolyte abnormalities in the critically ill small animal patient are both common and complex. The severity of Na and K disturbances remains a significant predictor of mortality in human ICUs [14]. Critical illness causes major stress on all the regulatory functions of the body, including those responsible for normal electrolyte balance. The stress of sepsis, acute renal failure, impaired perfusion, multiple organ dysfunction syndrome and the administration of vasoactive agents all affect the neuroendocrine system that controls serum electrolyte balance. Many therapies directed at maintaining vital organ function directly or indirectly affect these regulatory systems. Assessment of the patient electrolyte status can be further complicated by the infusion of electrolyte‐containing fluids and parenteral and enteral nutrition. Appropriate and timely diagnostic and monitoring procedures are required for early intervention and to improve patient outcome. The electrolyte status of the ICU patient will have an impact on every body system, with emphasis on the neurological, muscular, renal, cardiovascular, gastrointestinal, acid–base, and hematological functions. The underlying diseases, as well as the therapeutic interventions, place every ICU patient at risk for electrolyte disorders. Often one electrolyte abnormality can lead to abnormalities with the other electrolytes. Important information helping to recognize a problem, define the cause, and identify the effects of electrolyte disorders can be obtained through the history, physical examination, point of care (POC) testing, clinicopathological testing, diagnostic imaging, and monitoring tools. The history will begin with the signalment (age, sex, breed). Breed‐associated problems that can affect the electrolytes should be considered, such as pseudohyperkalemia found in Shar Pei, Akita, Japanese Shiba Inu and Korean Jindo [15] or Addison’s disease (causing hyperkalemia, hyponatremia) in the Standard Poodle and Bearded Collie. Female dogs are affected by hypoadrenocorticism twice as often as males. The past medical history may identify diseases such as diabetes mellitus, hyper‐ and hypoadrenocorticism, hyper‐ and hypothyroidism, renal disease, and various cardiovascular diseases that can manifest with acute and chronic electrolyte disorders (Tables 6.1, 6.2, and 6.3). A history of polyuria and polydipsia, gastrointestinal (GI) disturbance, neuromuscular changes (such as muscle weakness, fatigue, muscle tremors or twitches), seizure activity, change in mentation or behavioral changes, generalized weakness or difficulty breathing can suggest a cause or effect of an electrolyte problem. Historical problems such as high salt intake, protracted vomiting or diarrhea, severe hyperthermia, adipsia or hypodipsia, rapid ventilation, chronic nasal discharge or urinary obstruction should direct evaluation of serum Na. A list of prescribed and over‐the‐counter medications is important to identify any drugs that can have an impact on the electrolyte status. Table 6.1 Common disorders of sodium and potassium in the small animal ICU patient. ECF, extracellular fluid; ICF, intracellular fluid; SIADH, syndrome of inappropriate ADH secretion. Table 6.2 Common disorders of ionized calcium and magnesium in the small animal ICU patient. ECF, extracellular fluid; ICF, intracellular fluid. Table 6.3 Common disorders of corrected chloride and phosphorus in the small animal ICU patient. ECF, extracellular fluid; ICF, intracellular fluid; RBC, red blood cell. Physical and neurological examination findings that can be associated with electrolyte alterations in the ICU patient are summarized in Table 6.4. The severity of these clinical signs is often attributable to the magnitude and rapidity of the development of the disorder. Since electrolytes are critical for the flow of water and electrical charges across cell membranes, life‐threatening electrolyte problems will frequently be associated with fluid imbalances, or neuromuscular, vascular, cardiac or neurological abnormalities. Table 6.4 Common clinical signs associated with an excess or deficiency of the major electrolytes (Na, K, Ca, Mg, Cl, and P). The temperature, pulse rate and intensity, oral mucous membrane color, and capillary refill time provide a reasonable assessment of peripheral tissue perfusion and intravascular fluid balance. Skin turgor, mucous membrane and corneal moisture, and eye position within the orbit are used to estimate the hydration status (extravascular fluid balance) of the patient. An abnormal heart rate, pulse deficits, alterations in pulse intensity, and signs of poor perfusion can result from electrolyte abnormalities causing cardiovascular dysfunction. The presence of dementia, seizures, coma or tremors can be a neurological consequence of abnormal electrical impulses associated with electrolyte problems. Changes in muscle strength and tone (such as generalized weakness, ventral flexion of the head and neck, weak ventilator muscles) and motor activity may reflect electrolyte‐induced neuromuscular abnormalities. A minimum database is provided through POC in‐hospital testing and should include the packed cell volume (PCV), total protein (TP), blood glucose, blood urea nitrogen (BUN) electrolyte panel (Na, K, ionized Ca, Mg, Cl, P), blood lactate, acid–base status, coagulation profile, and urinalysis. A low PCV could be due to hypophosphatemia and red blood cell (RBC) lysis. Assessing the PCV and TP together might reflect overhydration or dehydration, each a potential cause or effect of a Na disorder. Alterations in blood glucose can be associated with changes in the plasma K concentration. Each electrolyte will play a role in the acid–base status of the patient, necessitating that the blood gas results be assessed in conjunction with the electrolyte panel results (see semiquantitative acid–base analysis in Chapter 7). Elevations in the BUN in conjunction with abnormal urine concentration, proteinuria, and cast formation can be an indication of renal disease, which can alter the excretion of all the electrolytes. Urine electrolytes, such as urine Na and Cl, have been reported to be helpful in discerning prerenal azotemia (low urine Na) from acute renal tubular damage (high urine Na), which can occur secondary to nephrotoxins or ischemia [16]. The results from the blood or serum electrolyte panel will provide the most important evidence of electrolyte disorders, but do not necessarily reflect the changes in total body (including intracellular and interstitial) electrolyte quantities. Normal electrolyte concentration will vary slightly among laboratories. A variety of techniques using ion‐specific electrodes can be utilized to measure serum electrolytes. Collection of blood samples into the correct blood collection tube is essential since the type of anticoagulant can falsely decrease or elevate electrolyte values. Blood or serum Mg concentrations will not reflect the important intracellular Mg concentration. Knowledge of the underlying disorder and any clinical signs attributable to an abnormal Mg concentration plays an important role in making the diagnosis of a Mg disorder. Serum Ca exists in three forms: 55% is ionized (physiologically active form), 35% is protein bound (primarily albumin and to a lesser extent globulin), and 10% is complexed to anions (citrate, bicarbonate, phosphate, or lactate). It is important to quantitate the active ionized Ca rather than the total Ca (which includes all three forms). Calcium is an important factor in blood coagulation, with alterations in ionized Ca a viable cause of both hypo‐ and hypercoagulable states. Serum Cl should be evaluated relative to the serum Na concentration. Proportional changes in Na and Cl reflect changes in water balance. Nonparallel changes in Cl relative to Na identify clinical disorders of Cl that affect acid–base balance (see Chapter 7). To account for changes in water balance, measured serum Cl should be corrected for changes in serum Na by the formula in Box 6.1. Normal corrected Cl is approximately 107–113 mEq/L in dogs and 117–123 mEq/L in cats but can vary with different laboratories and analyzers. Chloride is most commonly measured by potentiometry using an ion‐specific electrode. With this method, bromide, cyanide, and other halides are measured as Cl. Pseudohyperchloremia will occur in patients treated with potassium bromide. A complete blood count and serum biochemical profile will provide data pertaining to possible contributions of the internal organs to electrolyte abnormalities. An elevation in serum creatinine and BUN (along with the urinalysis) directs further investigation for kidney disease as the cause of fluid imbalance and abnormal serum electrolyte concentrations. Problems such as liver disease, pancreatitis or hypoadrenocorticism might be found as a cause of hypovolemia as well as altered electrolyte concentrations. Underlying problems known to be associated with electrolyte disorders are listed in Tables 6.1, 6.2, and 6.3. Additional testing is often required and could include fecal examination, serology for inciting pathogens (cause of SIRS‐related diseases), adrenal function testing (for hypoadrenocorticism, hyperadrenocorticism, or pheochromocytoma), thyroid panels, and diagnostic imaging. Fecal examination for parasites might find a cause for diarrhea and the resultant electrolyte changes. Pseudo‐hypoadrenocorticism (hyperkalemia and hyponatremia) is reported to occur with whipworm infections [17]. Survey thoracic and abdominal radiographs provide the initial diagnostic imaging data once the patient has been stabilized. Abdominal and thoracic ultrasound provides a more in‐depth assessment of organ structure and an evaluation of the size and structure of the adrenal glands, a possible source of electrolyte changes. The electrocardiogram (ECG) will provide a waveform image of the electrical activity of the cardiac conduction system which is generated by the electrolytes. Hyperkalemia will cause changes in the ECG. However, these changes are not predictive of the magnitude of the plasma K alterations, especially in cats (Figure 6.2). An example of ECG changes associated with hyperkalemia is shown in Figure 6.3. ECG changes are also seen with Ca disorders. The ECG hallmark of hypocalcemia is the prolongation of the QT interval due to lengthening of the ST segment. The exact opposite holds true for hypercalcemia [18]. Hypercalcemia produces a quicker ventricular repolarization and therefore shorter QT interval. Figure 6.2 Potassium levels and associated common electrocardiogram changes. Figure 6.3 Example electrocardiogram of a cat with hyperkalemia, recorded at 50 mm/sec; this ECG demonstrates atrial standstill (absence of P waves) with supraventricular escape beats (associated regular QRS and T waves present) at a rate of 140 bpm. The physical and neurological examination should be repeated at least twice daily for early detection of clinical signs of an electrolyte alteration before life‐threatening consequences occur. The electrolyte panel is assessed at least daily, or more frequently depending upon the urgency of the anticipated or diagnosed electrolyte disorder. Assessment of the fluid balance should be ongoing and can incorporate monitoring of body weight, central venous pressure (CVP), arterial blood pressure, echocardiography (diameter of vena cava or aorta), urine output, and blood lactate. The ECG is monitored for evidence of electrolyte‐related conduction disturbances and to assess the efficacy of any therapeutic interventions. Abnormalities in Na, K, Ca, Mg, Cl, and P concentrations can have serious consequences in the small animal ICU patient. The electrolyte disorder can occur as a direct result of the underlying problem, a consequence of the clinical signs associated with the underlying disease (such as vomiting, diarrhea, polyuria) or a complication of treatment (such as fluid therapy, drug administration). Blood Na concentration reflects the ratio of Na to water in the ECF and accounts for most of the osmotic particles in the serum. Normal serum Na is 139–154 mEq/L or mmol/L in the dog and 145–158 mEq/L or mmol/L in the cat. Note that the quantity of Na expressed in mEq/L is equivalent to the same quantity in mmol/L. Abnormalities in serum Na concentration are caused most commonly by changes in water balance. Total body water is estimated to be 60% of lean body weight. The distribution of this water is one‐third extracellular (intravascular and interstitial) and two‐thirds intracellular. The flow of water in and out of cells, particularly brain cells, is primarily responsible for the symptoms of both hyponatremia and hypernatremia. Clinical signs of Na disorders are nonspecific and may be difficult to separate from the clinical signs of the underlying disease. Central nervous system signs are most common with alterations in Na and can range from lethargy and weakness to ataxia, seizures, coma, and death. The severity of neurological dysfunction is related to the rapidity of onset and the degree of deviation from “normal.” The most life‐threatening consequence of Na disorders and their treatment is the excessive movement of water into or out of the brain cells in response to the osmotic shift that Na disorders cause in the interstitium and blood. A high interstitial Na (hypernatremia) will draw water out of the cells, causing cell shrinkage. In response to this, the cells will make idiogenic osmoles or osmolytes (taurine, glysine, glutamine, sorbitol, and inositol) to generate an intracellular osmolarity that is now similar to the extracellular hyperosmolarity. In contrast, a low interstitial Na (hyponatremia) will cause water to move from the interstitium into the cells where the osmolality is higher. This can lead to dangerous cell swelling and cerebral edema. The key to surviving a Na disorder is to manage clinical signs and restore the osmolar balance slowly. Hypernatremia is defined as a serum sodium concentration >155 mEq/L (>155 mmol/L) in dogs and >162 mEq/L (>162 mmol/L) in cats (see Table 6.4). One or more of the following mechanisms will be responsible for this hyperosmolar Na‐water imbalance: (1) Na gain greater than water gain, often due to the administration of hypertonic sodium‐containing solutions (such as hypertonic saline, sodium bicarbonate or hypertonic parenteral nutrition); (2) water loss greater than Na loss (such as osmotic diuresis, diuretics, gastrointestinal secretions); and (3) loss of solute‐free water (such as diabetes insipidus, adipsia, fever) (see Table 6.1). Normally, hypernatremia stimulates the thirst mechanism and renal water conservation through the action of ADH. In patients with intact thirst mechanisms and the ability to drink, serum Na levels can remain more normal despite possible ongoing water losses. However, several factors can contribute to the onset of hypernatremia in the ICU patient, including the inability to drink (such as orders for nil per os, vomiting, mental impairment, inability to swallow), impaired renal ability to resorb water (such as nephrogenic diabetes insipidus, renal medullary washout, glucosuria) and the administration of IV fluids and medications that affect water or Na concentrations (such as diuretics, Na‐containing fluids and drugs). Persistently elevated serum Na (>155 mEq/L or >155 mmol/L) in association with protracted hypotension portends a dismal prognosis in hospitalized hypernatremic human patients [19]. Possible causes of hypernatremia in the small animal ICU patient are listed in Table 6.1. Hypernatremia has been generally classified as hypovolemic (inadequate water intake or loss of water over Na), euvolemic (diabetes insipidus), and hypervolemic (intake of hypertonic fluids, hyperaldosteronism, salt poisoning). The more common causes are attributable to a relative lack of free water. Early symptoms of hypernatremia include lethargy and weakness, progressing to muscular rigidity, seizures, and coma. Untreated hypernatraemia (170–190 mmol/L) resulted in brain lesions demonstrating myelinolysis and cellular necrosis in rats and rabbits [20]. Normalization of the hypernatraemia over 4–24 hours resulted in cerebral edema, due primarily to failure of brain amino acids and idiogenic osmoles to dissipate as plasma Na is decreased to normal. Therapy for hypernatremia is first directed at correcting any concurrent perfusion deficits, and subsequently, correcting the serum Na with hypotonic fluids. An algorithm to guide the lowering of serum Na is presented in Figure 6.4. A four‐step plan for making the necessary calculations for the quantity of fluid required to lower the serum Na to the desired concentration is presented in Box 6.2 with sample calculations. The replacement method outlined estimates the Na‐lowering effect of possible choices of IV fluid formulations on the patient serum Na [21]. An advantage of this method is that Na values are known rather than estimated. Box 6.2 also lists the Na content of common fluid infusates. These formulas serve as a guideline, with frequent monitoring of serum Na and adjustments in fluid infusion made to meet the specific needs of the individual patient. Figure 6.4 Decision‐making algorithm for management of hypernatremia.
Electrolytes
Introduction
Specific functions of electrolytes
Diagnostic and monitoring procedures
History and physical examination
Hypernatremia
Hyperkalemia
Free water loss
Fever
Heat stroke
Adipsia
Primary hypodypsia
Diabetes insipidus
Central
Renal
Water loss > Na
Diuretics
Intrinsic renal disease
Post obstructive diuresis
Nasogastric suction
Osmotic cathartics
Cutaneous burns
Na gain > water
Salt ingestion
Sea water ingestion
Sodium bicarbonate
Hypertonic saline
Hyperalimentation
Sodium phosphate enema
Reset osmostat
Primary hyperaldosteronism
Essential hypernatremia
Impaired renal excretion
Prerenal azotemia
Thrombosis
Renal azotemia (acute, severe)
Postrenal azotemia
Uroabdomen
Reduced aldosterone level or response
Hypoadrenocorticism
Massive tissue injury
Systemic thrombosis
Tumor lysis syndrome
Post cardiac arrest
Severe heat stroke
Rhabdomyolysis
Acute metabolic acidosis
Diabetic ketoacidosis
Lactic acidosis
Post cardiac arrest
Release from intracellular stores
Hereditary disorders (such as Akitas)
Pseudohyperkalemia
Hyponatremia
Hypokalemia
Impaired water excretion
Hypoadrenocorticism
Diuretic therapy
Loss through bodily fluids
Vomiting
Diarrhea
Third body fluid space Na loss
Pancreatitis
Cutaneous burns
Hormonal dysregulation
SIADH
Hypothyroidism (myxedema coma)
Water overload
Hypotonic fluid administration
Congestive heart failure
Severe liver disease
Nephrotic syndrome
Advanced renal failure
Primary (psychogenic) polydipsia
Transcellular shift (ECF → ICF)
Acute metabolic alkalosis
Acute correction of acidosis
Insulin
Glucose
B2‐agonists (albuterol overdose)
Refeeding syndrome
Reduced intake
Inadequate K in parenteral fluids
Increased loss
Renal failure
Diuretic therapy
Osmotic diuresis
Post obstructive diuresis
Renal tubular acidosis
Primary hyperaldosteronism
Hyperadrenocorticism
Hypomagnesemia
Gastrointestinal loss
Vomiting
Nasogastric suction
Diarrhea
Hypercalcemia
Hypermagnesemia
Young growing animals
Malignancy
Renal failure
Hypervitaminosis D
Iatrogenic
Cholecalciferol rodenticides
Toxic plant ingestion
Antipsoriasis creams
Oral vitamin D supplementation
Granulomatous disease
Primary hyperparathyroidism
Hypoadrenocorticism
Idiopathic (cats)
Osteolytic diseases
Calcium administration
Lipemia
Renal insufficiency
Excessive Mg infusion or intake
Hypothyroidism
Hypoadrenocorticism
Milk alkali syndrome
Shift of K from ICF to ECF
Massive cell lysis
Hypocalcemia
Hypomagnesemia
Primary hypoparathyroidism
Secondary hypoparathyroidism
Neck surgery or trauma
Parathyroidectomy, thyroidectomy
Hypomagnesemia
Hypermagnesemia
Pancreatitis
Rhabdomyolysis
Renal failure
Eclampsia
Poor dietary intake Excessive P intake
Intestinal malabsorption
Ethylene glycol toxicity
Phosphate‐containing enemas
IV phosphate supplementation
Sepsis
Laboratory error
Hypoalbuminemia (total Ca)
Citrate toxicity
Hypovitaminosis D
Chelation with EDTA (transfusion)
Nutritional secondary
hyperparathyroidism
Gastrointestinal loss
Chronic diarrhea
Steatorrhea
Renal loss
Loop diuretics
Thiazides
Parathyroidectomy
Diabetic ketoacidosis
Hyperaldosteronism
Hyperthyroidism
Nephrotoxins
Eclampsia
Hyperchloremia
Hyperphosphatemia
Pseudohyperchloremia
Lipemic samples (colorimetric methods)
Potassium bromide therapy
Excessive loss of Na relative to Cl
Diarrhea
Excessive gain of Cl relative to Na
0.9% NaCl fluids
Hypertonic saline
Renal retention
Renal failure
Renal tubular acidosis
Drug induced
Spironolactone
Acetazolamide
Age of sample (release from RBCs)
Young growing animals
Renal
Acute or chronic renal failure
Pre‐ and postrenal azotemia
High phosphorus intake
Dietary, poor‐quality protein
Phosphate enemas
Vitamin D toxicity
Hypoparathyroidism
Intracellular release of P
Tissue trauma
Tissue necrosis
Tumor lysis syndrome
Hemolysis
Rhabdomyolysis
Hypochloremia
Hypophosphatemia
Pseudohypochloremia
lipemic samples (titrimetric methods)
Excessive loss of Cl relative to Na
Gastric fluid losses
Loop diuretics
Thiazide diuretics
Chronic respiratory acidosis
High Na intake compared to Cl
Bicarbonate
Penicillins
Inadequate intake
Dietary deficiency
Malabsorption
Hypovitaminosis D
Translocation from ECF to ICF
Alkalosis
GlucoseInsulin
Bicarbonate
Renal
Renal tubular defects
Diuretics
Eclampsia
Electrolyte change
Considered abnormal
Clinical Signs
Hyponatremia
Dog <140 mEq/L
Cat <142 mEq/L
Nonspecific central nervous system abnormalities: lethargy, weakness, ataxia, seizure, coma, death
Hypernatremia
Dog >155 mEq/L
Cat >162 mEq/L
Nonspecific central nervous system abnormalities: lethargy, weakness, ataxia, seizure, coma, death
Corrected hypochloremia
Dog <107 mEq/L
Cat <117 mEq/L
Signs are nonspecific and more likely related to the underlying disease process or changes in acid‐base status
Corrected hyperchloremia
Dog >113 mEq/L
Cat >123 mEq/L
Signs are nonspecific and more likely related to the underlying disease process or changes in acid base status
Hypokalemia
Dog, cat <3.5 mEq/L
Muscle weakness (skeletal muscle), ventroflexion of the neck (cats), rhabdomyolysis (severe), impaired ventilation (when severe), gastrointestinal ileus/atony, arrhythmias
Hyperkalemia
Dog, cat >5.5 mEq/L
Signs are attributable to the underlying disorder and brady‐ or tachyarrhythmias may be immediately life threatening resulting in signs of poor cardiac output; muscle fasciulations, gastrointestinal signs and polydypsia may also occur
Hypocalcemia
ionized
Dog <5 mg/dL <1.2 mmol/L
Cat <4.5 mg/dL <1.1 mmol/L
Behavioral changes, abnormal/stiff gait, panting, facial rubbing, muscle spasm/fasciculation, tetany, and seizures. Hyperthermia (secondary to increased muscle activity), anorexia, vomiting, and diarrhea have also been reported. In human patients, laryngospasm or bronchospasm have been reported. Cardiac changes including hypotension, bradycardia, arrhythmias, and poor Ca‐mediated drug response
Hypercalcemia
ionized
Dog >11.5 mg/dL >1.5 mmol/L
Cat >10.5 mg/dL >1.4 mmol/L
Polyuria, polydipsia, anorexia, dehydration, lethargy, weakness, hypertension, seizures, and acute renal failure. Mineralization of soft tissues is possible when Ca (mg/dL) × P (mg/dL) product is >60
Hypophosphatemia
Dog, cat <2.4 mg/dL (0.775 mmol/L)
Muscle weakness, rhabdomyolysis, hemolysis, impaired platelet and white blood cell function
Hyperphosphatemia
Dog, cat >6.0 mg/dL (>1.92 mmol/L)
No primary clinical signs, but signs associated with hypocalcemia or renal failure may be noted
Hypomagnesemia
Dog <0.7 mmol/L (<1.7 mg/dL)
Cat <0.41 mmol/L (<1.0 mg/dL)
Hypomagnesemia is commonly associated with other electrolyte abnormalities, including hypokalemia, hyponatremia, hypocalcemia, and hypophosphatemia. Signs ascribed to hypomagnesemia include cardiac arrhythmias, muscle weakness, muscle fasciculations, altered mentation, esophageal motility disorders, respiratory muscle paralysis, dysphagia, dyspnea, ataxia, seizures, and coma
Hypermagnesemia
Dog >1.2 mmol/L (>2.92 mg/dL)
Cat >1.25 mmol/L (>3.04 mg/dL)
Concomitant hypocalcemia, hyperkalemia, or uremia may exaggerate the symptoms. Patients demonstrated vomiting, bradycardia, hypotension, decreased mentation, hypothermia, weakness with generalized flaccid muscle tone with absent patellar and palpebral reflexes
Point of care testing
Clinicopathological testing
Diagnostic imaging
Monitoring methods
Electrolyte disorders
Sodium disorders
Hypernatremia
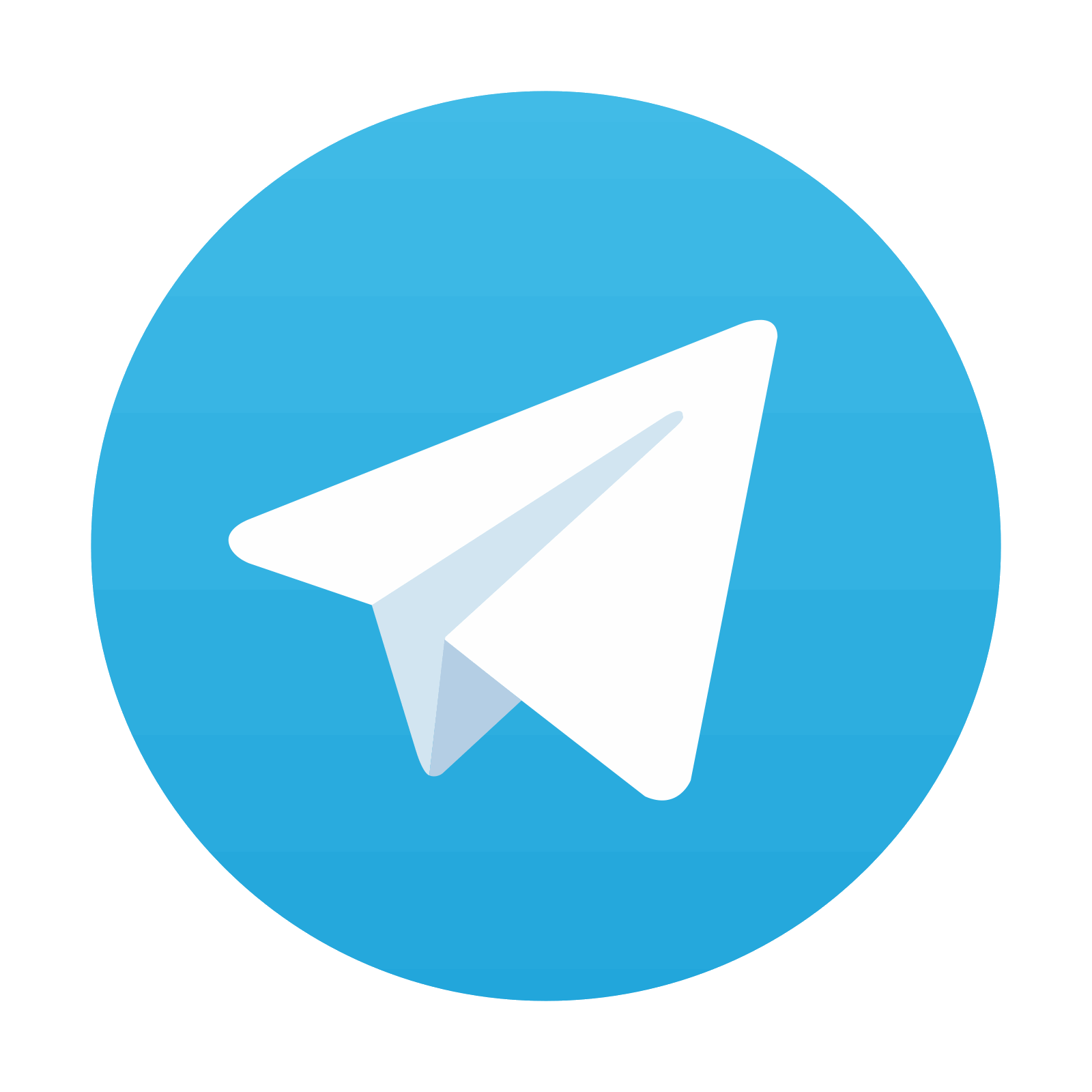
Stay updated, free articles. Join our Telegram channel
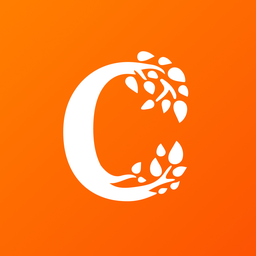
Full access? Get Clinical Tree
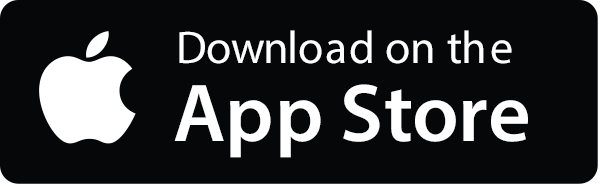
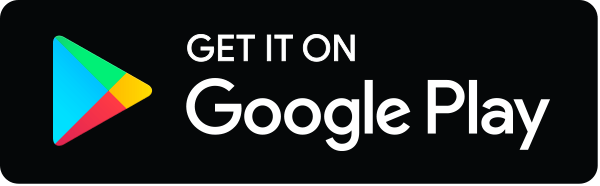