CHAPTER 4 Adesola Odunayo University of Tennessee, Knoxville, Tennessee Albumin has been found to be a nonspecific marker of critical illness [1]. The albumin level alone is of limited use in reaching a diagnosis for the patient. However, it has been found to be an important prognostic indicator of morbidity and mortality in critical veterinary and human patients. Unfortunately, the simple replacement of an albumin deficit has not been found to consistently improve survival. This suggests that the complex mechanisms underlying the alteration in serum albumin are of equal importance. A change in serum albumin will result in a corresponding change in the intravascular colloid osmotic pressure (COP). The COP has therefore also been demonstrated to be a prognostic indicator of pulmonary edema and mortality in human patients, with a low COP associated with an increased morbidity and mortality [2]. Albumin is the main protein of blood plasma, being small with a molecular weight of 65 000–69 000 daltons in the dog and cat. Box 4.1 provides the normal serum albumin concentration for those species. Highly water soluble, this elliptical shaped and flexible molecule has a strong net negative charge. It is made in the liver, with only 20–30% of the hepatocytes producing albumin at any one time. It is not stored, so cannot be released on demand. The primary factor controlling the rate of production is a change in the COP (also called oncotic pressure) and the osmolarity within the extravascular liver spaces [3]. Albumin synthesis is proportional to the hepatic interstitial COP. During maximal stimulus for production, the rate of synthesis increases only 2–3‐fold. Albumin is catabolized in or adjacent to the vascular endothelium of tissues at a daily rate similar to the daily rate of production. Breakdown of albumin involves uptake into endocytotic vesicles after binding to endothelial surface membrane scavenger receptors. These receptors are widespread in the tissues [4]. The vesicles then fuse with lysosomes for degradation within the endothelial cells. Figure 4.1 depicts the major contributing factors responsible for the plasma albumin concentration. Figure 4.1 Mechanisms of albumin gain, loss, and distribution. The quantity of albumin in the plasma is provided through synthesis by the hepatocytes or by administration of exogenous albumin or fresh frozen plasma (FFP) which contains albumin. Albumin will leak through the endothelial glycocalyx layer when the interendothelial gaps permit and contribute to the interstitial concentration of albumin (also known as the “albumin pool”). Interstitial albumin will move into the blood through the lymphatics. Albumin loss from the body occurs through catabolism, renal or intestinal loss or loss through hemorrhage or exudation (such as burns, wounds, effusions). Source: modified from Margarson M, Soni N. Serum albumin: touchstone or totem? Anesthesia 1998:53,789–803. Albumin is primarily an extravascular protein, with the largest quantity (grams) found in the interstitium (also known as the “albumin pool”). However, due to a wider distribution, the interstitium has a lower concentration (g/L) of albumin than the plasma. There is normal circulation of albumin between the intravascular space and the interstitial space through the lymphatic vessels. The movement of albumin through the capillary walls is called transcapillary escape, and is determined by the capillary, subglycocalyx, and interstitial free albumin concentrations, capillary permeability, solvent and solute movements, and electrical charge across the capillary wall [5]. Lymphatic flow is dependent upon interstitial fluid pressure, intrinsic lymphatic pumping, and external compression of the lymph vessels by muscle contraction, arterial pulsation, and body movement. The role of albumin is complex and multifaceted, and includes: Referred to as a molecular “taxi,” albumin binds water, cations (such as calcium (Ca++), copper (Cu++) sodium (Na+) and potassium (K+)), fatty acids, hormones, bilirubin, thyroxine (T4), and drugs. Though it has a strong negative charge, it can bind weakly to both cations and anions. There are four discrete binding sites on one albumin molecule, with ligands competing at a single site or altering their affinity for a remote binding site [7]. Drugs that compete for binding at a single site can displace one another, affecting drug concentration and potency. The binding of a drug to albumin will greatly affect the delivery of the drug to tissue sites, and the metabolism and elimination of the drug. Roughly 65–80% of the normal COP results from the presence of albumin, due to both its strong oncotic effect and negative charge. Colloid osmotic pressure is the force generated when two solutions with different concentrations of colloids (macromolecules, primarily albumin) are separated by a semipermeable membrane [8]. Sodium ions are noncovalently bound to albumin, contributing to the osmotic effect of that protein molecule; this is known as the Gibbs–Donnan effect [9]. The total oncotic pressure of an average capillary is about 21–25 mmHg in the dog and cat, with albumin contributing approximately 78% of this oncotic pressure. The remainder of intravascular COP is due to the concentration of globulins, fibrinogen, and hemoglobin, and, to a lesser degree, red blood cells (RBCs). Classically, the mechanism of transvascular fluid exchange has been described by Starling forces [10] (Box. 4.2), which include the capillary pore size (interendothelial cell space) and the hydrostatic pressure and oncotic pressure differences across the capillary endothelial barrier. The discovery of the endothelial glycocalyx layer (EGL) has led to the proposal of a revision of the Starling principle, often termed the glycocalyx model [11] (Figure 4.2). Some of the major differences between the original Starling principle and the glycocalyx model are listed in Table 4.1. Figure 4.2 Schematic comparing the Starling forces model of capillary fluid movement to the glycocalyx model. The arrows represent the directional movement of water, with thicker arrows representing greater force or flow in the direction of the arrow under normal circumstances. Capillary hydrostatic pressure (HP) is a result of blood pressure, cardiac output, and intravascular fluid volume. The interstitial tissues have HP that opposes the capillary HP but does not reverse the fluid dynamics until there is a significant increase of interstitial pressure, such as with large quantities of interstitial edema. The HP plays a similar role in both models. The colloidal osmotic pressure (COP) is primarily generated by albumin in both models. The Starling model has the intravascular COP opposed by the interstitial COP. When plasma albumin concentration is normal, the driving force of COP retains fluid in the vasculature. When intravascular COP is higher than the interstitial COP, water will move from the interstitium into the intravascular space. The glycocalyx model shows that the COP force opposing the intravascular COP is due to the presence of albumin in the subglycocalyx space, with the amount of albumin in this space determined by the porosity of the glycocalyx “meshwork” and the charge of the glycocalyx layer. In both models, the size and type of molecule that leaves the capillary are dependent upon the reflection coefficient of the vessel, the type of interendothelial junction (shown as X), and the charge. In the glycocalyx model, fluid re‐enters the vasculature through the lymphatics rather than directly from the interstitium. Table 4.1 Differences between the original Starling principle and the glycocalyx model of capillary fluid dynamics. COP, colloid osmotic pressure; EGL, endothelial glycocalyx layer; HP, hydrostatic pressure; ISF, interstitial fluid; IV, intravascular. Adapted from Woodcock TE, Woodcock TM. Revised Starling equation and the glycocalyx model of transvascular fluid exchange: an improved paradign for prescribinb intravenous fluid therapy. Br J Anaesth. 2012;108(3):384–94. The EGL is a web of membrane‐bound glycoproteins and proteoglycans positioned on the luminal side of the endothelial cells throughout the vasculature [12]. It has a net negative charge and acts as a macromolecular sieve [13]. It is semipermeable to anionic macromolecules, such as albumin, and when healthy, impermeable to RBCs and molecules greater than 70 000 daltons. The EGL regulates vascular permeability, influences blood cell‐vessel wall interactions, affects rheology, and controls the vascular microenvironment [14]. Because it lies on top of the vascular endothelial cells, the EGL creates a subglycocalyx intercellular space that has its own COP established by albumin within that space. The oncotic forces, in this model, are only set up across this endothelial surface layer on the luminal aspect of the endothelial cell, not across the whole capillary wall [15]. While intravascular and subglycocalyx albumin is still a major component of COP and fluid movement, the interstitial albumin concentration does not affect transcapillary fluid movement in this model. Breaks within the interendothelial cell junctions constitute the primary pathway for transvascular fluid filtration from the capillary. Albumin has an oncotic role across the EGL and the COP difference opposes but does not reverse transcapillary flow. In this model, fluid does not enter the capillary from the interstitium (except in the intestines, brain, and kidneys) but instead by way of lymphatic deposition of fluid into the venous system. Figure 4.2 provides a schematic summary of the Starling forces and glycocalyx model of transcapillary fluid dynamics. As a free radical scavenger, the sulfhydryl groups of albumin will scavenge reactive oxygen and nitrogen species, such as superoxide, hydroxyl, and peroxynitrite radicals. Albumin can also limit their production by binding free Cu++, which is known as an accelerant in free radical production [16,17]. Many critical small animals patients have lost albumin as a consequence of their underlying disease but present to the ICU with a normal initial blood albumin value and COP. This can be due to dehydration as well as redistribution of albumin from the interstitial “albumin pool” into the blood through the lymphatics. This can delay the recognition of albumin and COP disorders until serious consequences have occurred. A marked decrease in the COP can eventually result in interstitial or pulmonary edema and arterial hypotension causing poor tissue perfusion [2,18,19]. The administration of crystalloid fluids can dilute blood albumin concentrations, raise capillary hydrostatic pressure, and lower intravascular COP, making therapy an important additional inciting factor. Albumin is also a negative acute‐phase protein, meaning that hepatic production of albumin drops when systemic inflammation is present. The administration of various drugs can cause an increase or decrease in blood albumin (Table 4.2). It is important to identify and monitor patients that are likely to have a problem affecting albumin concentrations and the COP to minimize complications and maximize therapy. Table 4.2 Drugs and plasma albumin. NSAID, nonsteroidal antiinflammatory drug. Diagnostic testing to identify disorders of albumin and the COP should begin with the patient history, physical examination, and the minimum laboratory database done by point of care (POC) cage‐side testing. Additional clinicopathological testing, diagnostic imaging, and equipment‐based monitoring can provide insight into the cause and consequences of alterations in these important parameters. The history begins with the signalment (age, sex, breed). Age‐related changes in albumin values cause younger and geriatric animals to have a lower normal albumin concentration (see Box 4.1). Problems that can result in the loss of albumin may have a breed predilection, to include proteinuria in breeds such as Doberman Pinscher, Beagle, Collie, and Labrador Retriever; protein‐losing enteropathy in breeds such as Yorkshire Terrier, Basenji, Soft‐coated Wheaten Terrier; and liver disease in breeds such as Bedlington Terrier, Doberman Pinscher, and Labrador Retriever. Past medical problems might identify gastrointestinal (GI) or urinary tract signs compatible with protein‐losing disease. A complete review of the diet is done to find a nutritional source for albumin alterations. It is important to identify any possible exposure to drugs or toxins that could affect albumin concentrations or become potentially toxic due to changes in the albumin concentration. Table 4.2 provides a list of common drugs that are affected by albumin. The history might also reveal soft tissue swelling, labored breathing, polyuria, diarrhea, abdominal distension, and other clinical signs that can be related to alterations in blood albumin and COP (Table 4.3). Table 4.3 Organ systems that are affected by low COP and common clinical signs (from development of edema). The physical examination incorporates the vital signs (temperature, pulse rate, respiratory rate, and effort) with the physical peripheral perfusion parameters (heart rate, mucous membrane color, pulse intensity, capillary refill time) to evaluate blood flow to the peripheral organs. Hypovolemia due to low COP can lead to poor perfusion, and eventually dehydration if fluid intake has been inadequate. Hydration is assessed with skin turgor, eye position within the orbit, and mucous membrane and corneal moisture (see Chapter 2). Other physical findings indicative of hypoalbuminemia and a low intravascular COP can include cavitary effusions or tissue edema. Abdominal effusions are common and can manifest as abdominal distension, reduced bowel sounds, and short or shallow breaths due to increased pressure on the diaphragm. Fluid waves might be felt during abdominal palpation or ballotment, but may require large volumes. Pleural fluid accumulation is less common from this cause but can produce an elevated respiratory rate and effort and muffled lungs sounds. Pulmonary edema may cause respiratory distress with a rapid, shallow breathing pattern and moist lung sounds heard on auscultation. Patients with evidence of heart disease (murmur, gallop sounds or arrhythmias) may have underlying heart pathology, which can exacerbate pulmonary edema due to fluid intolerance and low COP. Heart murmurs can also be heard due to severe alterations in blood rheology associated with anemia or low blood protein. Edema of the intestines may result in poor GI function, altered normal borborygmi, vomiting or diarrhea. Peripheral edema is recognized first in body areas with thin skin, low muscle mass, and few fat stores, such as around the Achilles tendon or intermandibular space. Conjunctival edema (chemosis) is an early sign of fluid intolerance, followed quickly by subcutaneous fluid accumulation around the head and neck and the distal limbs. Other causes of interstitial edema or effusion such as increased hydrostatic pressure, right‐sided heart failure, portal hypertension, neoplasia or vasculitis should also be considered as a possible cause. Assessment of the patient for improvement or resolution of clinical signs associated with alterations in albumin and COP (hypotension, edema, pleural effusion, ascites, and respiratory distress) is an important monitoring tool throughout resuscitation and definitive treatment. The minimum laboratory database blood samples should ideally be drawn prior to fluid resuscitation. The POC tests will include the packed cell volume (PCV), total protein (TP), blood glucose, blood urea nitrogen (BUN), blood gas analysis, electrolyte panel, coagulation profile (prothrombin time, activated partial thromboplastin time), platelet number estimation, and urinalysis. A serum or plasma refractometer reading can provide a TP, which is a combination of albumin plus globulin and other blood proteins. A separate biochemical assay is required to determine the precise albumin and globulin concentration. An elevation in TP more commonly represents reduced plasma water but can be a consequence of an elevation in globulins. A low TP generally reflects a low albumin, with or without a loss of globulins, and can be found with dilution of blood with nonprotein‐containing fluids, loss of protein from the vasculature or lack of blood protein production. Synthetic colloids are not demonstrated on the refractometer and will dilute the concentration of albumin and other proteins [1]. Refractometer readings should be interpreted in light of evaluation of other microhematocrit levels, including PCV, serum color, and buffy coat; this may provide further insight into the underlying disease process. The BUN may be elevated with proteinuria from kidney disease or low with liver cirrhosis or failure, each a potential cause of hypoalbuminemia. Hyperglycemia can alter the glycocalyx layer of the vasculature, increasing the permeability of the glomerular capillary wall to albumin [20]. A large proportion of serum calcium is bound to albumin in the blood. Measuring the ionized portion of calcium will allow for a more accurate assessment of serum calcium concentrations. Blood albumin concentrations can also affect the acid–base status of the patient. Albumin acts as a weak acid, with hypoalbuminemia therefore having an alkalinizing effect with an increase in the base excess. Increases in blood albumin are associated with an acidifying effect. Treatment for either acid–base derangement involves specific therapy for the underlying disease. See Chapter 7 for further information. Platelet numbers may decline and coagulation times increase with disseminated intravascular coagulation (DIC), a common consequence of systemic inflammatory response syndrome (SIRS) diseases, to include sepsis. Increased vascular permeability leads to hypoalbuminemia. The transcapillary escape of albumin implies an interendothelial gap size of at least 69 000 daltons (size of albumin molecule). Antithrombin (AT), an important endogenous anticoagulant, has a smaller molecular weight (58 000 daltons) and will extravasate in a similar fashion. Loss of AT from the circulating blood can be a marker of a risk for thrombosis. The urinalysis is evaluated for proteinuria as evidence of a protein‐losing nephropathy and urine specific gravity (USG) as evidence of renal urine concentrating ability, which may indicate underlying renal disease. Administration of synthetic colloids may artificially raise urine specific gravity and should be considered when evaluating USG in critically ill animals. The urine sediment is examined for signs of a urinary tract infection as the cause of proteinuria or a focus of inflammation. Blood for a complete blood count (CBC) and serum biochemical profile is drawn prior to fluid resuscitation and submitted for analysis. Leukocytosis and leukopenia can each be a consequence of systemic inflammation or sepsis. The biochemical profile is evaluated for evidence of underlying diseases that cause disorders of albumin and COP. Table 4.4 provides a list of diseases that can cause changes in plasma albumin. The serum albumin concentration will be reported. Hyperalbuminemia is almost always associated with dehydration, but other causes must be considered. Hypoalbuminemia will be due to decreased production, transcapillary escape into tissues, albumin loss from the body, dilution by nonalbumin‐containing fluids or a combination of these mechanisms. Hypercholesterolemia, hypoglycemia, decreased BUN and prolonged clotting times, with or without elevated liver enzymes, are strongly suggestive of severe liver disease as the cause of hypoalbuminemia. Table 4.4 Common disorders causing an alteration in serum albumin and colloid osmotic pressure. SIRS, systemic inflammatory response syndrome. Hypoalbuminemia is consistent with a low COP in patients that have not been treated with natural or synthetic colloids. The COP can be calculated using serum albumin or total protein values; however, this method has been found to provide an inaccurate assessment of COP in the critically ill patient [21–24]. The gold standard for assessing COP in human and veterinary patients is to directly measure the COP of plasma, serum or whole blood with the colloid osmometer, a bench‐top membrane transducer instrument (Wescor Inc., Logan, UT). The normal value for measured COP in the dog is 21–25 mmHg and 23–25 mmHg in the cat [23,25]. Additional testing might be required to find the underlying cause of albumin alterations, such as serology for infectious pathogens, culture and susceptibility of urine or fluids, urine protein:creatinine ratio, drug concentrations, bile acids, and histopathology of intestinal biopsies. Analysis of fluid collected from thoracic or abdominal effusions will determine whether the fluid is a transudate (resulting from increased hydrostatic pressure and/or decreased COP) or exudate (resulting from inflammation) (see Table 4.5). Table 4.5 Differences in composition and characteristics between a transudate and an exudate. Diagnostic imaging begins with plain thoracic and abdominal radiographs. Thoracic radiographs are evaluated for pulmonary, cardiac or pleural space abnormalities. The presence of pleural fluid will direct centesis for diagnostic sampling and relief of pleural pressure. The presence of pulmonary edema can be a consequence of hypoalbuminemia and a low COP, heart disease, acute lung injury or acute respiratory distress syndrome. Abdominal radiographs can demonstrate organ size, positioning, and shape as well as the presence of abdominal effusion. Ultrasound may provide a more detailed evaluation of organs for evidence of cysts, fluid, infiltrates, hemorrhage, edema or other anatomical abnormalities. The thoracic and abdominal focused assessment with sonography techniques (AFAST and TFAST) are useful in identifying free fluid; bedside ultrasound lung examination (VetBLUE) may also be useful to identify pulmonary edema or other pathology (see Chapter 10 (AFAST) and Chapter 8 (TFAST, VetBLUE)). The aspiration of abdominal or pleural fluid can be guided by ultrasound with the fluid evaluated by culture, cytology, and biochemistry as indicated. Endoscopic examination and mucosal biopies of the stomach, upper duodenum, colon or rectum may be indicated when a protein‐losing enteropathy is suspected. Advanced imaging such as a portovenogram, nuclear scintigraphy, computed tomography or nuclear magnetic resonance imaging may be indicated to better define the extent and nature of a suspected or confirmed abnormality. The selection of equipment‐based monitoring tools will be guided by repeated physical examinations. Signs of poor perfusion, dehydration, peripheral edema, abdominal distension, and changes in breathing rate and effort can suggest fluid extravasation due to hypoalbuminemia and a lowered COP. The refractometer is used to easily follow the trends of change in total protein. The results can indicate a likely rise or fall in COP when crystalloids have been used as the sole resuscitation and maintenance fluid. However, direct measurement of the COP with a colloid osmometer is required after the administration of synthetic colloids since they are not measured by the refractometer. Monitoring blood pressure and pulse oximetry can provide an early indication of pulmonary complications attributable to hypoalbuminemia and a low COP and show progress during treatment. Monitoring the blood pressure, blood lactate, and urine output can provide insight into the tissue perfusion of core organs. The electrocardiogram can help with early detection of cardiac edema or ischemia. Careful patient assessment using the Rule of 20 (at least twice daily) is important to rapidly identify and manage potentially life‐threatening complications arising from alterations in blood albumin and the intravascular COP. Changes in serum albumin are the result of pathological events, not the cause of them. Critically ill patients often have one or more underlying disease processes that contribute to alterations in blood albumin and ultimately a significant change in COP (see Table 4.4). Both factors can have an important impact on the outcome of the patient. A high serum albumin is primarily a reflection of dehydration and is typically resolved through fluid therapy. However, low serum albumin and the resultant decrease in COP can be a component of any critical illness. The resulting complications of hypoalbuminemia must be strategically managed for an optimal therapeutic outcome. Hypoalbuminemia is to be anticipated as a complication of any critical illness. After a major insult, the serum albumin inevitably decreases and tends to increase slowly as the patient recovers. A clear association has been found between the albumin level and the severity of insult [26] but it is unclear whether there is a cause–effect relationship or whether it is just a marker of serious disease [27]. Hypoalbuminemia is associated with increased complications and reduced survival in critically ill humans, dogs, and cats [28–32]. Each 1.0 g/dL (10 g/L) decrease in serum albumin was associated with a 137% increase in the odds of death, an 89% increase in morbidity, and a 71% increase in length of hospital stay in humans [29]. Hypoalbuminemia in critical illness can result from mechanisms that include: Loss of protein from the blood into the GI tract occurs due to mucosal disease with ulceration, obstruction of lymphatic flow, increased mucosal capillary permeability or a combination of these problems. Chapter 15 provides more information pertaining to the impact of GI disease in the critical patient. Proteinuria occurs as the result of increased permeability of the glomerular filtration barrier, composed of the glomerular endothelial cell, the basement membrane, and the podocyte. Abnormalities in one or more of these components can result in proteinuria and albuminuria. The presence of protein in the renal tubule will stimulate inflammation and fibrinogenesis in the proximal tubular epithelial cells, contributing to the progression to end‐stage renal disease [34]. Chapter 13 provides options for the diagnosis and treatment of proteinuria. The resultant hypoalbuminemia contributes to the morbidity and mortality in both GI and renal disorders. The vascular endothelium is one of the earliest targets for inflammatory mediators in SIRS diseases and sepsis [35]. The EGL is damaged by TNF‐alpha, IL‐6, complement, and bacterial lipopolysaccharides. Cytokines, proteases, histamine, and heparinase released in inflammation further degrade the EGL. The transcapillary escape of albumin has been demonstrated in septic patients [36]. The loss of circulating albumin accompanies the loss of the glycocalyx, lowering the COP and increasing fluid extravasation [37]. Low intravascular COP in trauma patients correlated well to EGL degradation and thrombin loss, which subsequently affected vascular permeability and coagulation [38]. These changes can have a devastating impact on the intravascular fluid volume and oxygen delivery to the tissues [39]. Hypovolemia is an anticipated consequence and can lead to inadequate oxygen delivery, increased lactate production, decreased adenosine triphosphate (ATP) production, cell membrane breakdown, cellular death, organ failure, and death. Even a very minor intravascular volume deficit could lead to ischemia of splanchnic organs in the critical veterinary or human patient [40]. The onset and outcome of acute lung injury and acute respiratory distress syndrome are closely associated with hypoalbuminemia. The clinical signs resulting from low albumin are most frequently due to the accompanying decrease in intravascular COP. The signs can be subtle or quite dramatic. Common signs of low COP in critical patients are listed in Table 4.3 and determination of albumin deficit is noted in Box 4.3.
Albumin and colloid osmotic pressure
Introduction
Concept
Original Starling principle
Glycocalyx model
Composition of intravascular volume
Plasma and cellular elements
Glycocalyx, plasma, red cell distribution volume
Separation of intravascular from interstitial volume
Capillaries with high COP (proteins) within and ISF with lower COP (protein)
EGL semipermeable to anionic proteins with higher COP within and interendothelial clefts below with lower COP (proteins)
Site of fluid absorption
Along the capillary, magnified at venous end based on IV and ISF COP concentration difference
Continuous capillaries exhibit no absorption; fluid returned to vasculature via lymphatics
Important forces
Transendothelial (HP) pressure differences and plasma‐interstitial COP difference
Transendothelial pressure difference and plasma‐subglycocalyx COP difference; COP of ISF is not a direct determinant of transendothelial flow
Effect of raising COP within the capillary
Enhances absorption and shifts fluid from interstitial space to plasma
Reduced transcapillary flow but does not cause absorption
Effect of subnormal capillary pressure (HP)
Net transcapillary absorption increases plasma volume
Transcapillary flow approaches 0; any autotransfusion is acute and limited in quantity
Effect of supranormal capillary pressure (HP)
Net transcapillary filtration increases ISF volume
Transcapillary flow is proportional to transendothelial pressure differences (when COP difference is maximal)
Effect of infused colloid solution and isotonic crystalloid
Colloid distributed through plasma volume; crystalloid through the extracellular volume (IV and ISF)
Initially same as Starling’s model. At supranormal HP, colloid preserves COP, raises HP, increases transcapillary flow. At supranormal HP isotonic crystalloid raises HP, lowers COP and increases transcapillary flow more than colloid. At subnormal HP, colloid increases plasma volume and crystalloids increase intravascular volume but transcapillary flow is low for both fluids
Drugs that increase plasma albumin concentrations
Drugs that decrease plasma albumin concentrations
Drugs that bind to albumin
Anabolic steroids
Androgens
Growth hormone
Insulin
Albumin concentrates
Fresh frozen plasma
Frozen plasma
Whole blood
Crystalloid fluids
Synthetic colloid fluids
NSAIDS
Sedatives
Antiepileptics
Digoxin
Antibiotics
Anticoagulants
Steroids
Furosemide
Glucose
Thyroxine
Amino acids
Vitamins
Folate
Vitamin D
Diagnostic testing and monitoring
History and physical examination
Organ system affected
Clinical signs
Cardiovascular system
Poor perfusion and arrhythmias; pericardial effusion with jugular vein distension
Gastrointestinal system
Vomiting, diarrhea, poor absorption of nutrients, weight loss, abdominal effusion
Respiratory system
Increased respiratory rate and effort, decreased lung sounds if effusion, wet lung sounds if pulmonary edema
Nervous system
Brain: mental depression, dementia, obtundation, stupor, coma
Spinal cord: ataxia, paresis or paralysis
Integument
Peripheral edema/weeping skin lesions
Eyes
Chemosis
Point of care testing
Clinicopathological testing
Hypoalbuminemia
Hyperalbuminemia
Decreased production
Hepatic disease
Acute inflammation
Sepsis
Malnutrition
Increased loss
Protein‐losing enteropathy
Protein‐losing nephropathy
Hemorrhage
SIRS diseases
Vasculitis
Sepsis
Parvoviral enteritis
Hemorrhagic gastroenteritis
Burns
Exudative wounds
Trauma
Decreased intake
Malnutrition
Anorexia
Malabsorptive diseases
Decreased plasma water
Adipsia
Dehydration from any cause
Excessive production/poor catabolism
Severe infection
Hepatitis
Overdose of cortisone
Chronic inflammatory disease
Congestive heart failure
Kidney disease
Cancer (hepatic carcinoma)
Hypoadrenocorticism
Iatrogenic
Albumin administration
Other drugs such as insulin
Transudate
Exudate
Low cellularity
Intermediate to high cellularity
Watery component of plasma, often clear in appearance
Large molecules in solution (i.e. fibrinogen), often cloudy in appearance
Low protein content, <2.5 g/dL
High protein content, >2.9 g/dL
Unlikely to clot
More likely to clot
Low specific gravity, <1.012
High specific gravity, >1.020
Low albumin or low fluid/serum protein ratio, <0.5
High albumin or high fluid/serum protein ratio, >0.5
Diagnostic imaging
Equipment‐based monitoring
Disorders of albumin and colloid osmotic pressure
Hypoalbuminemia and low COP
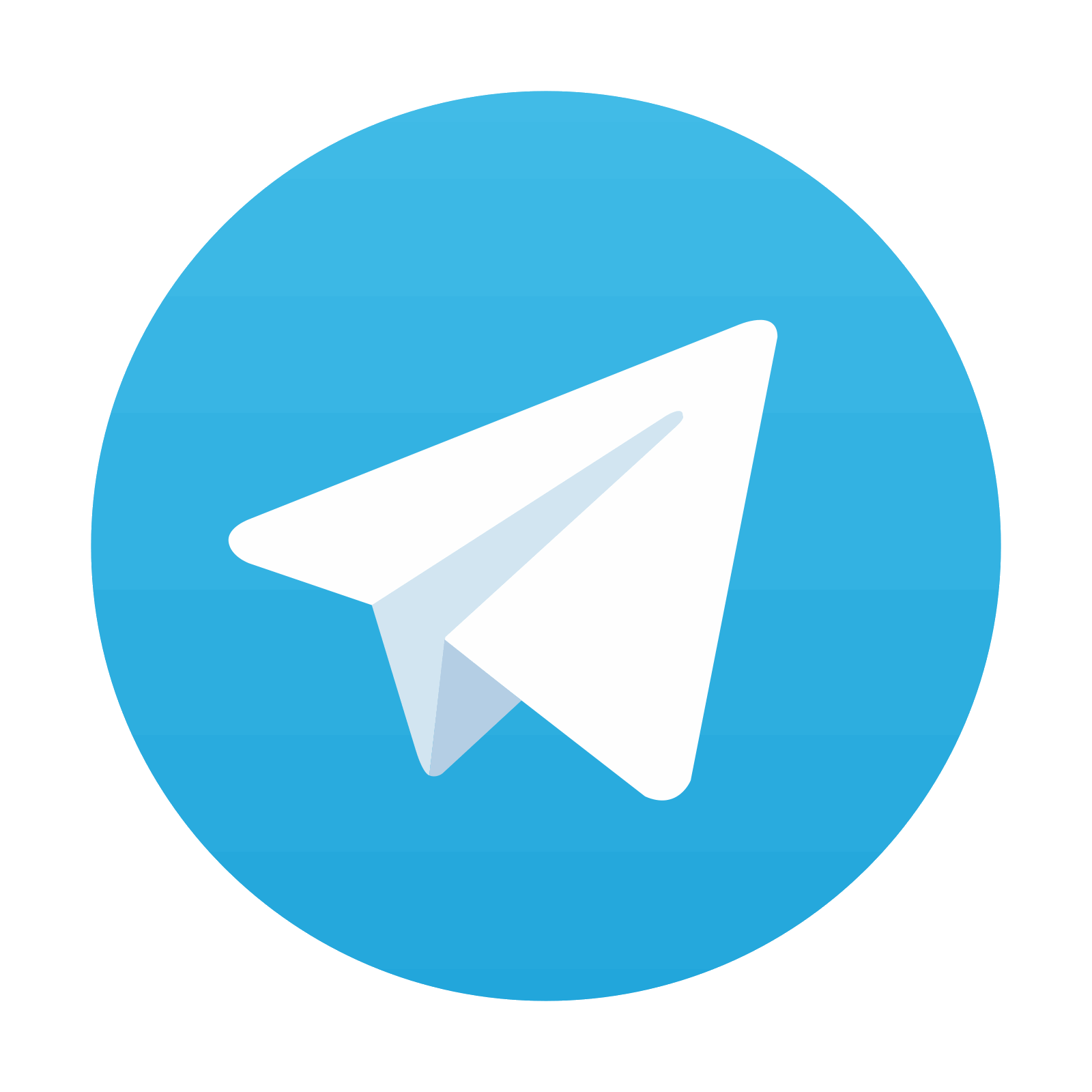
Stay updated, free articles. Join our Telegram channel
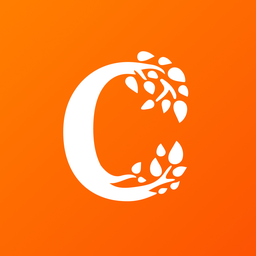
Full access? Get Clinical Tree
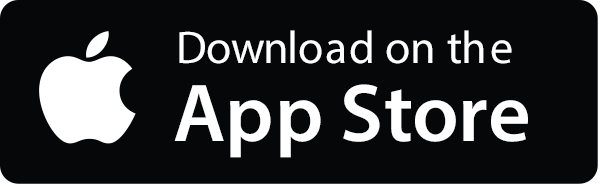
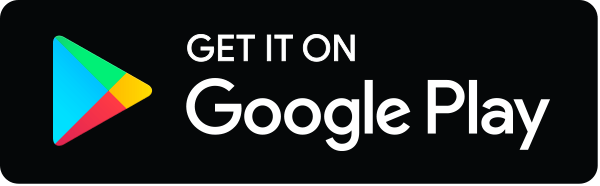