CHAPTER 2 Rebecca Kirby1 and Elke Rudloff2 1 (Formerly) Animal Emergency Center, Gainesville, Florida 2 Lakeshore Veterinary Specialists, Milwaukee, Wisconsin Water is the most essential nutrient of the body. It is the transport medium that brings oxygen, solutes, and hormones to the interstitium and delivers waste products to the liver, kidneys, and lungs for breakdown and excretion. In the interstitial space, water facilitates movement of these substances between the capillary and the cell. Within the cell, water provides a medium for organelles and for expansion of the cell membrane. Dissipation of heat occurs through the evaporation of water. Sixty percent of the body mass (0.6 L/kg) is made up of water [1]. The total body water (TBW) is partitioned into segments according to how water is contained. There are two major compartments where water is located: the intracellular compartment (66% TBW, 0.4 L/kg) and the extracellular compartment (33% TBW, 0.2 L/kg), which are separated by the cell membrane. The extracellular fluid (ECF) compartment is further divided by the vascular membrane into intravascular and interstitial compartments. The interstitial fluid compartment makes up 75% of the ECF compartment (25% of the TBW), and the intravascular fluid compartment makes up 25% of the ECF compartment (8% of the TBW) (see Figure 2.1). Figure 2.1 Schematic of body water dynamics at the level of the capillary. The Starling’s forces across the capillary membrane favor the retention of water in the capillary due to a higher concentration of colloid molecules (green circles) in the intravascular space compared to the subglycocalyx space. The Pc is the major force favoring fluid movement from the capillary into the interstitium. It is a result of CO and BP. The stroke volume is dependent on the preload, thereby a driving force behind the Pc. Sodium is the primary component of the osmotic pressure in the capillary and interstitium, and is freely permeable to the capillary membrane. It is not freely permeable to the cell membrane (yellow arrow). Potassium provides the major intracellular osmotic force that holds water within the cell. Water is freely permeable to the cellular membrane. The lymphatics pass through the interstitial space and remove water and solutes from the interstitium (creating a negative interstitial pressure) and return them back to the intravascular space via the thoracic duct. The intracellular space contains 66% of the TBW, the interstitium 25% of the TBW and the intravascular space 8% of the TBW. Red cylinder, capillary; blue square, cell; blue arrows, water movement; blue background, interstitial fluid; sodium, yellow circle; potassium, orange circle. CO, cardiac output; COP, colloidal osmotic pressure; EC, endothelial cells; GC, glycocalyx layer; HR, heart rate; Pc, capillary hydrostatic pressure; SV, stroke volume; SVR, systemic vascular resistance; TBW, total body water. Water moves freely across all membranes (cell and vascular) that separate fluid compartments. Two principles, osmosis and the modified Starling’s equation, govern how water remains within or moves between compartments. Osmosis is the process by which fluid diffuses through a semipermeable membrane from a solution with a low solute concentration to a solution with a higher solute concentration until there is an equal solute concentration on both sides of the membrane. Water moves across the cell membrane between the intra‐ and extracellular compartments by osmosis. Small molecular weight solutes on one side of the semipermeable plasma membrane hold water because they generate osmotic pressure. The primary solutes that generate intracellular osmotic pressure are potassium and magnesium, and the primary solutes that generate extracellular osmotic pressure are sodium, chloride, glucose, and urea [2,3]. When the concentration of solutes changes on one side of the cellular membrane and is no longer equal across the membrane, an osmolar gradient is produced. Water will move from the compartment with the lower solute concentration to the compartment with the higher solute concentration. Any sudden change in osmolality in the intravascular and interstitial compartment can affect the movement of water across the capillary membrane. The capillary membrane is freely permeable to small solutes and water, so any increase in solute concentration (osmolarity) in either extracellular compartment will be short‐lived. The components of the modified Starling’s equation produce the forces that dictate water movement within the extracellular space (Jv) across the capillary membrane, defined by the following formula [4,5]. The hydrostatic (hydraulic) pressure within the vessel (Pc) is generated by cardiac output (CO) and systemic vascular resistance. Hydrostatic pressure within the interstitium (Pis) is produced by the presence of water within the collagen fibrils and fibroblasts. Colloid osmotic pressure (COP; π) is the osmotic force generated by protein particles (such as albumin, fibrinogen, and globulins) that attract water. The COP within the plasma (πc) is opposed by the COP within the subglycocalyx space (πseg, virtually zero under normal conditions) (see Figure 4.2 in Chapter 4). The movement of colloid particles across the endothelial membrane will be affected by the osmotic reflection coefficient (σ), and the movement of water and particles will be controlled by the filtration coefficient (kf). In normal tissues, the lymphatic circulation is continuously removing fluid and particles from the interstitium (Q lymph), creating a slight negative interstitial pressure that promotes continuous flow of fluid and particles from the intravascular space through the interstitium into the lymphatic system which deposits them back into the intravascular space (via the thoracic duct). The major contributing forces affecting capillary fluid dynamics are illustrated in Figure 2.1. Fluid balance refers to the state of TBW homeostasis. Euvolemia and euhydration refer to a state of normal water content in the intravascular and interstitial fluid compartments, respectively. Hydration is the taking in of water, and describes the clinical state of TBW. Dehydration is a reduction in water content, when intake through food and water is less than output lost in feces, urine, sweat, and respiratory vapor. Clinically, the term dehydration is used to reflect the state of insufficient water content in the interstitial space. Overhydration is a condition of excess water content in the interstitial and intracellular spaces. Transmembrane ion pumps and channels regulate the movement of solutes and water in and out of the cell, maintaining cellular and organelle integrity. The primary active pump for solute transport across the cell membrane is the sodium‐potassium pump. Additional membrane pumps and channels regulate the movement of calcium, hydrogen, chloride, magnesium, glucose, and amino acids. These membrane transport systems are present in every cell in every organ, and many require energy to function. Energy required to drive transmembrane ion exchange is supplied by cleavage of adenosine triphosphate (ATP). In contrast to the 38 ATP molecules that are produced during aerobic metabolism, anaerobic metabolism produces lactate and only two ATP molecules per glucose molecule. Oxygen and glucose are transported from the intravascular space to the cell through a fluid medium. Carried by hemoglobin to the capillaries, oxygen normally diffuses with great ease through the capillary membrane and interstitial space, then into the cells, most of which are located less than 50 micrometers from a capillary. Perfusion is the delivery of oxygen to the tissues. The conduit for fluid that transports oxygen and glucose is the vascular system. The heart serves as the pump of the conduit. Oxygen delivery is the product of arterial flow and arterial oxygen content. Hemoglobin concentration and hemoglobin oxygen saturation are the prime components of arterial oxygen content, with dissolved oxygen content in plasma being a minor component (see Chapters 8 and 10). Arterial flow is a product of CO and systemic vascular resistance. Cardiac output is a product of myocardial contraction and heart rate. Venous return, as defined by the Frank–Starling law of the heart, increases the stretch of the heart chambers (preload), which increases the force of myocardial contraction. Factors influencing venous return to the heart include mean circulatory filling pressure, right atrial pressure, and resistance of the arteries (see Chapter 11). Blood flow is also influenced by pressure differences and compliance within the vascular circuit as well as the viscosity of the fluid medium. Extrinsic and intrinsic regulation of the cardiovascular system also affects blood flow to the tissues. Intrinsic metabolic autoregulation affects local organ blood flow, and is influenced by oxygen availability and the accumulation of metabolic byproducts. Continuously produced and utilized, adequate ATP production becomes heavily dependent on oxygen availability during high energy output states of critical illness. Optimum ATP production, therefore, depends on both oxygen delivery (DO2) to the cell and oxygen utilization (VO2) by the cell. Other than oxygen supplementation and maintaining adequate hemoglobin concentration, reestablishing and maintaining intravascular fluid volume supports maximum oxygen delivery. Compensatory neuroendocrine responses are initiated for restoring blood volume and meeting metabolic demands occurring during decreased CO states and increased ATP demands. Hormonal mechanisms control the volume and distribution of TBW and involve the kidney and the brain [6–9]. These mechanisms are continuously adjusting the amount of water retained and lost in response to fluids lost and taken in, balancing TBW. Loss of extracellular water with little or no solute (hypotonic fluid loss) results in an increase in plasma osmolality. This increase is detected by osmoreceptors which send an afferent signal to the hypothalamic paraventricular nuclei and cause the release of antidiuretic hormone (ADH) [10,11]. ADH increases the concentration of aquaporins in the renal collecting ducts, facilitating water reabsorption and therefore concentrating the urine. An increase in plasma osmolality is also detected by the thirst center (near the supraoptic and preoptic nuclei in the anteroventral region of the third ventricle), stimulating the sensation of thirst and an increase in water intake [12]. In the critically ill or injured patient, the mechanisms that balance fluid compartments are challenged and can become impaired. Water deficits result in altered body temperature regulation, neurological dysfunction, electrolyte imbalances, and hypovolemia causing reduced organ perfusion, acute kidney injury, and eventually, death [13,14]. Excess water can result in altered ventilation and lung function, gastrointestinal dysfunction, electrolyte imbalances, and cerebral edema [15]. Movement of water between compartments and into and out of the body can occur rapidly, and is not tolerated in critically ill patients. The normal compensatory responses might not occur as quickly as needed and can be incomplete, inappropriate or ineffective due to end‐organ dysfunction. Frequent assessment of the fluid balance in the critical small animal patient is necessary throughout hospitalization to identify the cause(s) of fluid imbalance, and to make appropriate adjustments in the treatment plan. Monitoring the fluid balance of a patient should occur on a regular basis since changes can be very drastic over relatively short periods of time. The history and physical examination provide initial insight into problems that can affect or be affected by an altered fluid balance. In hospital point of care (POC) testing, clinicopathological laboratory tests, diagnostic imaging, and monitoring procedures are each assessed in light of the physical status of the patient. Historical and presenting problems reported by the owner that can be associated with or affect assessment of water balance include vomiting, diarrhea, respiratory problems, blood loss, trauma, toxin exposure, fever, nasal discharge, heart failure, alterations in water intake and urine output, and exposure to environmental heat extremes. A list of prescribed and over‐the‐counter medications should be reviewed for drugs that might affect water balance (such as diuretics or vasodilators). Recently administered medication (such as ketamine or atropine) can alter mucous membrane moisture without being a reflection of TBW changes. The physical examination will identify abnormal parameters associated with fluid imbalance, in particular those associated with perfusion and hydration. Parameters that reflect peripheral perfusion include heart rate, mucous membrane (MM) color, capillary refill time (CRT), pulse quality, level of consciousness, and body temperature. Reduction in intravascular volume leads to clinical signs of hypovolemic shock. A progression of shock manifests in alterations in perfusion parameters, listed in Table 2.1. Rectal temperatures represent peripheral body temperatures and are an indirect indicator of the status of peripheral blood flow. Redistribution of blood flow from the periphery to the core with vasoconstriction can give a differential core‐to‐peripheral temperature (see Chapter 17). Table 2.1 Physical examination and measured parameters that reflect peripheral perfusion in the normal state and during the stages of shock. The presence of three or more variables attributable to a particular stage of shock supports a diagnosis of that state of perfusion. bpm, beats per minute. Clinical signs of compensatory shock in the dog can be easily overlooked. The signs of hyperemic mucous membranes, tachycardia, rapid capillary refill time, and normal to increased arterial blood pressure should not be interpreted as normal. The arterial blood pressure is being maintained at the expense of the increased heart rate and mild vasoconstriction. The cat does not typically manifest a compensatory shock response since this stage lasts only seconds to minutes after the initiation of shock in the cat [16]. The state of hydration is in continuous flux, and there is no single parameter that reflects hydration status accurately. Physical examination findings and laboratory indices are used to estimate current hydration status [17]. Physical parameters used to assess hydration include mucous membrane and corneal moisture, skin turgor, and eye position within the orbit (Table 2.2). Two important qualifications must be considered: (1) acute changes in tissue hydration may not be evident on physical examination, since there has not been time for compensatory fluid shifting (underestimating the percentage of dehydration), and (2) older or emaciated animals have poor skin turgor and sunken eyes in their orbit unrelated to their hydration status (overestimating the percentage of dehydration). Table 2.2 Physical examination findings and the associated estimated percent dehydration (interstitial fluid deficits). Up to 90% of acute changes in body mass can be attributed to a change in TBW, so that in the critically ill patient, a 1 kg change in body weight may be equivalent to a 1 L change in TBW [18–22]. However, due to third body fluid space accumulation (in the abdominal or pleural spaces, or intestines or uterus) or tissue edema, a change in body weight may not occur even though there is loss of fluids from the intravascular or interstitial space(s). Weighing patients, particularly small patients, every six hours is recommended and is a simple monitoring tool to use when assessing trends of change in fluid balance. Any systemic inflammatory response syndrome (SIRS) disease (such as sepsis, pancreatitis, trauma, immune‐mediated disease, neoplasia) will have increased capillary permeability as part of the syndrome that can result in fluid extravasation. This can lead to complications associated with hypovolemia, interstitial edema, and third body space fluid accumulation. Abdominal distension, reduced bowel sounds, and short or shallow breaths due to increased pressure on the diaphragm can provide physical evidence of a large accumulation of abdominal fluid. Fluid waves might be felt during abdominal palpation. Pleural fluid accumulation can produce an elevated respiratory rate and effort, and muffled lungs sounds. Pulmonary edema may cause respiratory distress with a rapid, shallow breathing pattern and moist lung sounds heard on auscultation. Edema of the intestines may result in poor gastrointestinal function, altered borborygmi, vomiting or diarrhea. Areas of the body that have thin membranes, low muscle mass, and/or lack of fat will demonstrate fluid accumulation first. Conjunctival edema (chemosis) is an early sign of fluid intolerance, followed quickly by subcutaneous fluid accumulation around the common calcaneal tendon, intermandibular space, head and neck, and the distal limbs. Box 2.1 lists common clinical signs of overhydration. Causes of interstitial edema or cavitary effusion unrelated to increased capillary permeability include oliguric kidney failure, right‐sided heart failure, and portal hypertension. Hypovolemia may or may not be associated with these problems. The minimum POC laboratory database that should be assessed before and during fluid therapy includes the packed cell volume (PCV), total protein (TP) measured by refractometer, creatinine, blood glucose, plasma lactate, serum electrolytes, acid–base status, coagulation times, platelet estimate, and urine specific gravity (USG). Following the trend of change over time is necessary since the infusion of fluids and other forms of therapy will result in changes that may require intervention. Loss and gain of extracellular water can result in hemoconcentration (increased PCV and TP) or hemodilution (decreased PCV and TP). Hemorrhage should be considered when the PCV and TP are reduced, although patients with acute hemorrhage can have normal or increased PCV as a result of sympathetic‐induced splenic contraction releasing red cells back into the circulation. In that situation, a reduction in the initial TP can be a tell‐tale sign of hemorrhage in a patient with a compatible history. An initial low TP without hemorrhage might reflect hypoalbuminemia and fluid shifts due to a loss of intravascular COP. As fluid resuscitation and rehydration are performed, hemodilution will result in a decrease in both PCV and TP. Decreased DO2 due to inadequate blood flow caused by hypovolemic shock can result in anaerobic metabolism. Hyperlactatemia, metabolic acidosis, and increased base deficit are usually associated with conditions causing tissue hypoxemia. Intracellular volume changes cannot be identified on physical examination. The clinician must rely on changes in the effective osmolality of ECF (primarily recognized by changes in sodium concentration) to mark changes in cell volume. Hyponatremia will be associated with movement of water from the ECF into the intracellular fluid (ICF) compartment, and a subsequent increase in intracellular volume. Hypernatremia will be associated with a decrease in intracellular volume (see Chapter 6). Changes in blood glucose can affect water balance. Hyperglycemia increases plasma osmolarity and can result in increased extracellular water if a patient receives or takes in fluid. Hyperglycemia also induces glycosuria, diuresis, and water loss. A significant loss of TBW can occur when water losses exceed intake, and can result in hypovolemia, dehydration, and hypernatremia (see Chapter 5). Urine specific gravity is a reflection of the kidney’s functional ability to concentrate urine. The USG can be used during assessment of fluid balance. A low USG in the patient receiving intravascular fluid (IVF) support can be difficult to interpret by itself. An inappropriately low USG in the clinically dehydrated patient, or one with azotemia, may indicate altered kidney function. If the USG is normal to increased in the patient receiving fluid therapy, fluid losses may be exceeding fluid intake and additional fluids may be warranted. Abnormal clotting times and platelet estimate can cause internal hemorrhage and signs of hypovolemia, necessitating careful titration of intravascular fluids to prevent a sudden increase in hydrostatic pressure and exacerbation of bleeding (see Chapter 9). Patients with hypercoagulable conditions may benefit from fluid therapy to prevent capillary stasis. An automated complete blood count with a manual differential count, a serum biochemical profile, and urinalysis should be reviewed for changes that might impact fluid balance and fluid therapy. Evidence of liver failure (low BUN, hypoalbuminemia, hypoglycemia, increased coagulation times, alterations in serum cholesterol) can be a cause of portal hypertension and a loss of intravascular COP with fluid extravasation into the intestinal interstitium and peritoneal cavity. An elevation in creatinine and reduced USG support renal disease, which can result in water and electrolyte imbalances. Hypertension and fluid retention will occur if oliguria or anuria develops. Hypoalbuminemia lowers intravascular COP and can potentiate fluid loss from the intravascular space (see Chapter 4). Significant proteinuria may signify altered glomerular function and, when severe, can result in hypertension, a relative oliguria, and interstitial edema. When investigating for causes of a solute‐free water imbalance, the serum osmolarity should be compared to the urine osmolarity. Normal serum osmolarity ranges from 290 to 310 mOsm in the dog and 290 to 330 mOsm in the cat [23]. Urine osmolarity can range between 161 and 2830 mOsm/L in normally hydrated dogs [24] and between 600 and 3000 mOsm/L in normally hydrated cats [25]. As the serum osmolarity rises, the urine osmolarity should also rise when the kidney works to conserve water. When there is an excess of water in the body, the normal kidney will dilute the urine, eliminating extra body water. Ethylene glycol and other small molecular weight toxins can increase measured plasma osmolarity without increasing calculated plasma osmolarity. This will cause an increased osmolar gap and alter fluid distribution and balance. Many osmolar toxins can be tested for in commercial laboratories. Survey thoracic and abdominal radiographs can be examined for signs of intracavitary fluid accumulation. Loss of abdominal detail and abdominal distension on abdominal radiographs are signs suggestive of peritoneal fluid accumulation. Distended pulmonary vessels can be caused by increased pulmonary vascular pressure. Increased interstitial densities and alveolar lung patterns are consistent with fluid accumulation in the lung parenchyma. Pleural fissure lines and retraction of the lungs from the pleural wall with surrounding soft tissue opacity suggest thoracic fluid accumulation. Small caudal vena cava and heart sizes are compatible with hypovolemia (Figure 2.2). Figure 2.2 Lateral abdominal radiograph of dog with gastric dilation‐volvulus. Note the small size of the vena cava (white arrows) and the cardiac silhouette supportive of insufficient intravascular volume. Volume replacement is critical prior to induction of anesthesia. Ultrasonography is more specific than radiography for demonstrating peritoneal, pleural, and/or pericardial fluid accumulation, organ structure, tissue edema, vessel size and obstructions to blood flow. Thoracentesis or abdominocentesis for fluid retrieval and sampling can be guided using ultrasonography. Echocardiography can provide important information regarding the role that the heart might have in patients with hypotension or fluid imbalance. Hemodynamic monitoring can be invasive or noninvasive, continuous or intermittent, physical or biochemical. Perfusion parameters and vital signs should be routinely and repeatedly evaluated from the start of fluid resuscitation until the patient leaves the hospital. Heart rate can be assessed by pulse palpation, continuous ECG monitoring, direct cardiac auscultation, Doppler blood flow monitoring or by pulse oximetry. “Bounding” pulse quality can be associated with compensatory shock, anemia, or pain. Weak pulse quality can be associated with decreased CO from decompensatory shock or heart failure, vasodilation, or severe vasoconstriction. Body temperature is an indirect indicator of peripheral blood flow within the tissues. Pain, anxiety, and abnormal body temperature may influence sympathetic tone and should be taken into consideration when assessing perfusion parameters. Indications of successful resuscitation of perfusion deficits include a normalization of heart rate, improved pulse quality, CRT of 1–2 seconds, pink MM color, normalization of body temperature, and improvement in mentation. Periodic monitoring of arterial blood pressure, central venous pressure (CVP), oximetry, urine output, blood lactate, and venous blood gas can be performed in those patients requiring more extensive monitoring. Arterial blood pressure is a frequently monitored parameter for documenting hypo‐ and hypertension and assessing the success of fluid resuscitation. Arterial blood pressure is an indirect estimation of perfusion and can be a reflection of intravascular volume status when cardiovascular function is normal. Arterial blood pressure should always be assessed in conjunction with heart rate and other parameters of perfusion. Direct arterial blood pressure monitoring is considered to be the “gold standard” for blood pressure monitoring. Persistently unstable patients that require intensive monitoring may benefit from direct arterial blood pressure monitoring (see Chapter 3). This technique is invasive and requires skill and specialized equipment. It can be difficult to place an arterial catheter in the hypovolemic or vasconstricted patient, and the use of a transducer and special monitor is required. Indirect methods for monitoring arterial blood pressure utilize a Doppler or an oscillometric monitor. The indirect method of measuring arterial blood pressure is the most efficient and least expensive means for monitoring arterial blood pressure, but studies correlating indirect to direct methods have shown inconsistent results, especially in cats [26–31]. Indirect arterial blood pressure does not accurately reflect venous return or regional blood flow [32], so the trend of change in indirect arterial blood pressure in conjunction with improved physical perfusion parameters implies a positive response to therapy. Pain, anxiety, cardiac dysrhythmias, and hypothermia may result in blood pressure readings that do not accurately reflect intravascular volume status. Urine output volumes can provide a nonspecific estimation of perfusion to the kidneys. Normal urine production depends upon adequate renal blood flow and a mean arterial pressure >60 mmHg. Mean arterial pressures below this value can result in oliguria. A urine output >1–2 mL/kg/hour is ideal in the critically ill patient undergoing treatment. If the patient has a decreased urine output, one must evaluate for hypotension, postrenal obstruction, and acute kidney injury. Central venous pressure is used to assess right ventricular preload. Central venous pressure reflects intravascular volume status when there is normal right heart function and vascular tone with no obstruction to flow. The two main factors that will reduce CVP are hypovolemia and venodilation, and an increase in CVP following a fluid challenge may indicate a fluid‐responsive condition. There is controversy regarding the predictability of CVP monitoring for distinguishing fluid responders from nonfluid responders. This necessitates that the CVP be evaluated in light of the entire clinical picture [33–39]. Elevated CVP measurements can correlate with volume overload, right or left cardiac dysfunction, increased intrathoracic pressure or an increase in pulmonary arterial resistance [40]. The goal during fluid resuscitation of the hypovolemic patient is to achieve and maintain a CVP = 5–8 cm H2O. A brief description of how to set up and manually measure the CVP is provided in Figure 2.3. In‐line water manometers or pressure transducers are used to measure CVP. Figure 2.3 General equipment and patient positioning to measure the central venous pressure (CVP) through a central venous catheter using a water manometer. Placement requires the use of sterile technique and equipment. A central catheter is placed with the tip of the catheter located near the base of the heart (with jugular venous placement) or in the intrathoracic caudal vena cava (with femoral vein placement). A water manometer is attached to the central port of a three‐way stopcock. The outflow port is attached to a fluid extension set and the inflow port to an intravenous fluid infusion set attached to a bag of isotonic fluids. The water manometer port is closed, the inflow and outflow lines are filled with fluid. The outflow line is attached to the patient. The inflow line is closed to the patient and the water manometer is filled to at least 20 cmH2O. The apparatus can be held by hand during measurement or can be attached to a solid surface (as in the photograph). Each measurement should be taken with the patient and apparatus in the same position. The “0” point on the manometer should be at the estimated level of the right atrium of the patient. To measure the CVP, the fluid infusion port is closed. Time is allowed for equilibration which is when the fluid column stops falling and the meniscus of the fluid column in the manometer oscillates up and down with ventilation. Once the level is stabilized, the bottom of the meniscus is read at the CVP measurement. Alternatively, CVP can be measured with a transducer and electronic monitor instead of a manometer. Successful placement of arterial and jugular venous catheters for direct blood pressure (BP) and CVP monitoring is challenging in the hypovolemic and small patient, and may require cutdown techniques for vascular placement. The supplies needed for placing an arterial catheter are listed in Box 3.2a and the technique outlined in Box 3.2b in Chapter 3. A brief description of how to perform a jugular or cephalic vein cutdown for catheter placement is outlined in Box 2.2. A more detailed and complete description of techniques for obtaining vascular access can be found in the Further reading list at the end of this chapter. Lactate has proven its value as a significant variable associated with mortality, and is part of the Acute Patient Physiological and Laboratory Evaluation (APPLE score) for dogs and cats [41,42]. Data from canine studies of hemorrhagic shock [43,44] and critically ill and injured dogs [45–47] have demonstrated that an increase in initial plasma lactate is correlated with a worse prognosis after blood loss. More importantly, a lack of lactate clearance is associated with an increase in morbidity and mortality [48–57]. Associative data suggest that lactate normalization during resuscitation is a more powerful indicator of resuscitation adequacy than oxygen‐derived variables. The goal with fluid resuscitation of the patient with hyperlactatemia is to have a return of lactate to normal. Despite its usefulness in evaluating response to resuscitation efforts and as a prognostic indicator, serum lactate concentration is not a sensitive marker for identifying local tissue hypoperfusion. The hydration status should be monitored daily by evaluating change in the MM moisture, skin turgor, body weight, and PCV/TP. Clinical signs of dehydration and overhydration are listed in Table 2.2 and Box 2.1. Comparing fluid intake with fluid output at least once daily may identify a patient’s response to treatment and the need for adjustment in fluid therapy. In severely compromised small animal patients, advanced monitoring procedures may be indicated for obtaining additional clues to fluid balance status. Determination of TBW using isotope dilution and neutron activation analysis techniques is considered the “gold standard” but this has not been investigated in the critically ill patient [58]. Multifrequency bioelectrical impedance analysis is a method that has been used to identify acute fluid shifts in critically ill people and racing horses, but controversies exist on its reliability [59–61]. This procedure differentiates extracellular water or intracellular water from TBW by using the electrical properties of body tissues. Extracellular water is quantified using low‐frequency current, and TBW is quantified using the data from higher frequencies. Intracellular water can be assessed by subtracting the values measured between the two water compartments [62]. Pulmonary artery (PA) pressure, pulmonary capillary wedge pressure (PCWP), CO, and venous oximetry can be measured for additional information on problems with perfusion status. Venous oximetry can be performed using mixed venous blood (SvO2) from the PA catheter or distal jugular venous blood (ScvO2) from a central venous jugular catheter. Mixed venous hemoglobin oxygen saturation is considered more specific for global oxygen delivery and utilization compared to ScvO2; however, ScvO2 has been determined to be an accurate and sensitive marker of ongoing hemorrhage and a predictor of success of resuscitation in experimental models of canine hemorrhage [63,64]. In dogs with severe sepsis or septic shock related to pyometra, an admission ScvO2 < 52% carries an increased risk of death [65]. Considering dogs in the ICU, an ScvO2 < 68% is associated with an increased mortality risk [66]. Jugular venous hemoglobin oxygen saturation must be evaluated in light of other perfusion parameters, body temperature, cardiac function, and arterial oxygen concentration. If normalization of physical perfusion parameters does not correlate with a higher ScvO2, causes of a reduced PCV, PaO2, and CO need to be investigated, and inotropic therapy considered [67]. Pulmonary artery catheter placement is used to measure not only SvO2 but also pressures in the right atrium and PA as well as the PCWP. The PCWP reflects left ventricular diastolic pressure, and can be used during assessment of left ventricular function, CO, and fluid responsiveness. The predictability of PCWP in distinguishing fluid responders from fluid nonresponders and the lack of improving mortality with the use of PA catheters have resulted in a significant reduction in their use in human medicine [68–71]. The cost of equipment and supplies, the technical skills required to place and maintain PA catheters, the experience needed to interpret monitored parameters, and the risk to the patient have made the use of PA catheters uncommon in clinical veterinary medicine. This fluid balance monitoring technique is being overshadowed by newer imaging technology. Ultrasonography can be used to assess intravascular volume by measuring inferior vena cava (IVC) collapsibility and by observing the dynamic changes of the ventricles during systole and diastole. In people, the compliant IVC changes diameter with TBW changes and respiratory cycle [72,73]. A flat IVC diameter during inspiration (<2 cm) has been shown to be an indicator of poor prognosis in trauma patients and acute surgical patients [74]. The collapsibility index is defined as the difference of the IVC diameter measured 2 cm distal to the hepatic vein inlet during inspiration (IVCi) and expiration (IVCe) in relation to expiration value ((IVCi‐IVCe)/IVCe) [75–80]. In people, a collapsibility index >50% is supportive of noncardiogenic, nonobstuctive types of shock (such as hypovolemic, distributive) [81], and in spontaneously breathing patients with hypovolemia, a collapsibility index >42% can predict an increase in CO after fluid infusion [82]. This monitoring technique is still under evaluation in people, and has not yet been validated in animals. Echocardiography can be used to globally assess the heart for pericardial fluid and cardiac tamponade (end‐diastolic right ventricular and right atrial collapse). Myocardial contractility should also be evaluated, and characterized as being hyperdynamic, normal, decreased or severely dysfunctional. Hypovolemic and distributive shock can be associated with hyperdynamic myocardial function in the normal heart [83]. The cardiac chambers appear small and contractions are vigorous and hyperkinetic with the endocardial surfaces almost touching during systole (“kissing walls”) [84–86]. Newer transcutaneous pulse oximeters (PVI® on MasimoSET®, Masimo, Irvine, CA) may be able to provide additional information regarding cardiovascular dynamic variables that can be measured in patients that are being manually or mechanically ventilated. Variations in pulse amplitude or pulse intensity (PI) of the pulse pressure waveform during respiratory cycles (inspiration or expiration) have been associated with fluid responsiveness. The PI is calculated by indexing the infrared pulsatile signal against the nonpulsatile signal and expressing this number as a percentage. The plethysmography variability index (PVI) is automatically calculated with the following formula: A PVI >20% is suggestive of hypovolemia, and the hemodynamic instability will likely be responsive to fluid administration [87]. This method is still under investigation in veterinary medicine. Videomicroscopy with sidestream dark field imaging has also been used to evaluate blood flow in the membranes of the oral cavity. This method estimates total vessel density, proportion of perfused vessels, microcirculatory flow index, and perfused vessel density. It permits visualization of the microcirculation during low flow states, and following therapeutic interventions. This method has been used in anesthetized dogs [88,89] but requires a practiced skill in acquiring views, time for image analysis, and the patient must be very still (anesthetized, sedated or very ill) during image acquisition. Additional monitors for peripheral perfusion have been evaluated experimentally, but are not available as a bedside test or not commonly used in veterinary medicine. Tissue partial pressure of CO2 can be measured in highly vascular areas, including sublingual, brain, conjunctiva, skin, and intestinal surfaces. Hypoperfusion leads to an increase in tissue CO2 levels. The most common areas interrogated are the sublingual and gastric mucosal surfaces. Elevated tonometry values and gradients between mucosal and arterial PCO2 are associated with impaired perfusion. The use of tonometry has not become routine in human or veterinary medicine [90–93]. The most common and persistent problem in the management of the critical small animal patient is establishing and maintaining fluid balance. There is no single test or combination of tests that accurately and consistently identifies fluid balance disorders. Significant changes can happen rapidly and at any time, necessitating frequent patient reassessment and modification of the fluid therapy plan. While guidelines are provided below for establishing resuscitation and maintenance fluid therapy plans, wide variation can occur between patients, necessitating an individualized approach to fluid therapy. Too little and too much fluid can be catastrophic. An acute iatrogenic overexpansion of the intravascular compartment causes stretch of the atria and release of atrial natriuretic peptide (ANP), increasing renal water and sodium excretion [94,95]. However, before excess water can be eliminated, there is an immediate increase in intravascular hydrostatic pressure. A dilutional decrease in the intravascular COP results when the excess volume is from a crystalloid fluid infusion. These changes in Starling’s forces will favor the extravasation of fluids from the capillaries into the interstitial compartment, with tissue edema a potentially serious consequence. The most common cause of fluid overload is the injection of excessive intravenous or subcutaneous fluids. Other causes include an increase in total body sodium content with a subsequent increase in extracellular water, such as occurs with congestive heart failure, kidney failure, liver failure, and oliguria. The intravenous administration of hyperosmolar solutions (such as mannitol, hypertonic saline, diagnostic contrast medium), blood products, and medications dissolved in fluid can also cause an increase in intravascular volume. The severity of the clinical consequences of intravascular fluid excess is dependent on the organ(s) that are affected and their ability to handle excess fluid. The clinical signs commonly associated with intravascular fluid excess in the dog and cat are listed in Box 2.1. The urgency of treatment is guided by the severity of signs. Treatment of mild signs typically includes restriction of water intake and restriction or discontinuation of fluid administration. Moderate signs might improve with the administration of diuretics (such as furosemide, 1–2 mg/kg IV) and restriction or discontinuation of the intake of water, fluids, and sodium. Severe signs might require discontinuing fluid administration, the administration of diuretics and the use of vasodilators and positive inotropes to support cardiac output. Dialysis may be indicated if the patient is oliguric or anuric. If pulmonary edema caused by fluid overload is causing hypoxemia, then temporary mechanical ventilation may be necessary. Circulatory shock is a life‐threatening phenomenon of ineffective circulating volume. The most common cause of circulatory shock is loss of intravascular volume (hypovolemic shock) through mechanisms such as hemorrhage, vomiting, diarrhea, decreased water intake, increased metabolic activity, and/or third body fluid spacing. However, there can also be insufficient return of blood to the heart due to peripheral vasodilation (distributive shock) or obstruction of central venous blood flow (obstructive shock). In these two conditions, the blood volume can actually be within “normal limits” but is ineffective at maintaining tissue perfusion. Animals in shock with poor cardiac performance (cardiogenic shock) will also have a reduced CO but the circulating intravascular volume can be variable (high, low or normal). The hemodynamic signs manifesting for each of these forms of shock are essentially the same, but fluid resuscitation is usually only beneficial in patients with hypovolemic and distributive forms of shock. Intravascular fluid deficits cause a decrease in venous return and CO. A schematic of the basic mechanisms of hypovolemic shock in the dog and cat is presented in Figure 2.4. The renin‐angiotensin‐aldosterone system will cause an increased fluid retention by the kidneys. A release of ADH stimulates the renal conservation of water and sodium [96]. These mechanisms serve to increase intravascular volume and venous return and improve CO and arterial flow. Energy is required to sustain this compensatory mechanism, and an additional oxygen supply is required for energy production. Substrates required for cellular energy production are provided through the actions of the stress hormones (glucagon, growth hormone, cortisol, and epinephrine). Figure 2.4 Schematic of the mechanism of the shock response in the dog and cat. This simplistic representation shows the response in the dog in blue lines and boxes and the response in the cat in green lines and letters. A decline in cardiac output initiates the process, with decreased stretch of the baroreceptors sending afferent signals to the brainstem. There is an increase in sympathetic output in both the dog and cat, resulting in a release of norepinephrine and epinephrine which increases heart rate and contractility, and causes a mild vasoconstriction. This is the compensatory stage of shock. This is where the clinical signs diverge in the dog and cat. In the dog, there is suppression of the parasympathetic output and heart rate remains elevated, but in the cat, a vasovagal type response is elicited and a normal or slow heart rate develops. If the baroreceptors are still not stretched, more dramatic sympathetic stimulation occurs with shunting of the peripheral blood to the core circulation (dog and cat). This response is the early decompensatory (or middle) stage. Energy is required to maintain these compensatory responses. When energy becomes depleted, the rapid heart rate and vasoconstriction can no longer be maintained. The heart rate slows and vasodilation results. This is the decompensatory (or terminal) stage of shock. Centrally mediated hypothermia plays a role in the middle stage of shock in the cat, resulting in the triad of hypothermia, bradycardia, and hypotension. These natural neuroendocrine responses can be adequate to compensate for mild‐to‐moderate acute decreases in intravascular volume, and produce the compensatory stage of hypovolemic shock commonly recognized in dogs. Cats experience the compensatory stage, but it is short (seconds to minutes) in duration and rarely recognized. Clinical signs of compensatory shock in the dog (see Table 2.1) include hyperemic mucous membranes, tachycardia, rapid capillary refill time, and normal to increased arterial blood pressure, which should not be interpreted as normal. Maintaining arterial blood pressure occurs at the expense of an increased heart rate and mild vasoconstriction (both energy consuming). Should the natural neuroendocrine mechanisms be inadequate to restore baroreceptor stretch, should cardiac dysfunction exist, or should intravascular volume and systemic vascular resistance be inadequate in restoring oxygen delivery to the tissues, cardiovascular decompensation occurs. Continued low CO amplifies sympathetic stimulation, clinically manifesting as significant peripheral vasoconstriction and tachycardia. Selective vasoconstriction of the skin, mucous membranes, and splanchnic beds shunts arterial blood flow to preferred organs (heart and brain), maintaining blood flow to those vital organs as long as possible. Cellular oxygen and energy demands increase as vasoconstriction intensifies. Oxygen consumption becomes dependent on oxygen delivery, and anaerobic glycolysis results in lactate production and hydrogen ion release. This multilevel cellular dysfunction places the animal in the early decompensatory (middle) stage of hypovolemic shock. Clinical signs of this stage in the dog include tachycardia, pale mucous membrane color, prolonged capillary refill time, decreased jugular vein distension, hypotension, and oliguria (see Table 2.1). Cats with hypovolemic shock will present with a subnormal temperature, decreased heart rate, and low arterial blood pressure. In the cat, the neuroendocrine response to hypovolemia appears to promote vasodilation, hypothermia, and bradycardia. Rather than an inhibition of the parasympathetic tone, the cat experiences a response similar to a vasovagal reflex that blunts the typical tachycardic response. Centrally mediated hypothermia is a shock response in the cat that leads to a poor response of adrenergic receptors to the increased catecholamine release, augmenting vasodilation and bradycardia. Vasoactive substances produced due to local tissue hypoxia at the capillary level cause local vasodilation and increased capillary permeability, resulting in maldistribution of blood flow in the hypoxic tissue beds. When chemical mediators (cytokines) produced locally in hypoxic tissues enter the systemic circulation, they incite a systemic inflammatory response syndrome (SIRS). Significant vasodilation and damage at the endothelial lining resulting in increased capillary permeability further deplete intravascular volume. Redistribution of blood flow away from the heart and the brain occurs, leading to further hypoxic consequences and the stage of late decompensatory shock. This effect is compounded when central pathology blunts the typical compensatory response, intravascular volume loss is massive, earlier compensatory responses are ineffective or inadequately treated, or the insult is severe and overwhelming. The mitochondria are unable to produce enough ATP, and the ensuing circulatory collapse and insufficient arterial flow to the brain and heart cause these and other organs to malfunction, and eventually fail. Clinical signs of this terminal stage include a relative bradycardia, hypotension, substantially delayed (>3 sec) or no capillary refill time, white or cyanotic MM color, hypothermia, altered mentation, and anuria (see Table 2.1). A positive outcome is optimized with early recognition of the clinical signs, hemostasis when applicable, as well as adequate and timely fluid resuscitation that incorporates specifically selected resuscitation goals for the patient. Relative to fluid balance, three presumptions exist: volume overload is bad, inadequate volume is bad, and what we conclude about the fluid balance of the patient may be incorrect. It is important to realize that the presence of fluid responsiveness is not an absolute indication that fluids are necessary. The decision to administer fluid therapy must be supported not just by a proof of volume responsiveness, but also by a need for hemodynamic improvement. Fluid infusion per se is not always the correct therapy for hemodynamic instability. Cardiac performance should also be evaluated in animals with a compatible history, physical examination or disease process that suggests abnormal cardiac function (see Chapter 11) prior to infusing large quantities of fluid for resuscitation. Thoughtful consideration must be given to the amount and rate of fluids to be administered in terms of individual patient needs, the underlying problems, and the degree of risk associated with fluid administration when outlining a fluid therapy plan. The fluid therapy plan involves resuscitation, rehydration, and maintenance phases. Oxygen supplementation (see Chapter 8) and analgesic administration are part of the resuscitation process. It is best to titrate analgesics and sedatives to effect, as responses are variable and can be affected by underlying renal and hepatic dysfunction (see Chapter 19). For all types of pain, methadone (0.2–0.4 mg/kg IV q 2–6 hours), hydromorphone or oxymorphone (0.1–0.2 mg/kg IV), or fentanyl (0.3–0.5 μg/kg) can be titrated to desired analgesic effects with or without a sedative (such as diazepam or midazolam, 0.2 mg/kg IV). The choice of fluid(s) to administer will be based on the properties of the specific fluid, the needs of the patient, and any potential side‐effects that might negate the selection of that fluid. Crystalloid fluids are typically chosen as the foundation of fluid therapy, supporting both intravascular and interstitial volumes. The addition of synthetic colloids or blood products is often warranted when there are significant intravascular volume deficits, with the goal of extending the duration of intravascular volume expansion. Blood products can provide additional support through the administration of RBCs, albumin, and coagulation factors. A crystalloid fluid is a water‐based solution with small molecules that are osmotically active in the body fluids and freely permeable to the capillary membrane. The amount that remains in the vessel depends on Starling’s forces and the distribution of TBW (see Figure 2.1). The sodium concentration provides the greatest contribution to the osmolarity of the crystalloid solution. Convention has defined an isotonic fluid as one that has an osmolarity equal to that of erythrocytes and therefore does not affect the exchange of fluid across the erythrocyte membrane. A hypertonic fluid will decrease erythrocyte volume, and a hypotonic fluid will increase erythrocyte volume. Crystalloid solutions commonly administered to the dog and cat are listed in Table 2.3 with the sodium, potassium and calcium content, osmolarity, pH, and common indications for selection. Table 2.3 Common crystalloid solutions used in the critically ill dog and cat. Na+, sodium; K+, potassium; Ca++, ionized calcium. A hypotonic fluid has an osmolarity less than intracellular fluid, such as 5% dextrose in water (D5W) or half‐strength saline (0.45% saline). To prevent red cell lysis from the infusion of hypotonic fluid into the intravascular space, dextrose is added to provide enough osmotic pressure to prevent movement into the blood cells. As the dextrose is metabolized, however, the water travels into the interstitial then the intracellular compartments based on normal TBW distribution. Other solutions with solutes that are metabolized (such as partial parenteral nutrition fluids) can also be used to provide water. Hypotonic fluids are not used during the resuscitation or rehydration phase but can be used to reestablish solute‐free water deficits in treating the patient with hypernatremia, after resuscitation and rehydration have occurred. When serum sodium values are greater than 170 mEq/L in the presence of altered mentation, replacement of free water is indicated (see Chapter 6 for guidelines). The solute‐free water deficit is calculated and replaced over 24–48 hours. The rate of sodium decline should be no more than 0.5–1 mEq/h to avoid rapid neuronal osmotic shifts and cell swelling. The volume of fluids is administered in addition to maintenance requirements. Hypertonic fluids such as 7% and 23% saline have more osmotically active particles per unit volume than intracellular fluid. The dose recommended for rapid intravascular volume resuscitation is 4–6 mL/kg of a 7% saline solution. After administration, water moves through osmosis from the interstitial and intracellular compartments into the intravascular space. There is a rapid increase in intravascular volume until Starling’s forces bring the equilibrium back across the capillary membrane. This may be mitigated when hypertonic saline is combined with hydroxyethyl starch (one part 23% hypertonic saline with three parts hydroxyethyl starch, 4–6 mL/kg). Adequate interstitial and intracellular fluid must be available for intravascular volume expansion to occur after the intravenous administration of a hypertonic solution. Hypertonic saline has been reported to produce a mild inotropic effect [97,98] with mild systemic and pulmonary vasodilation [99], improve immune function [100–102], and rapidly expand intravascular volume [103]. Hypertonic saline can also be administered to reduce intracranial pressure in brain‐injured patients [104]. In dogs with a hemoperitoneum, 7% hypertonic saline (HTS) combined with 6% hydroxyethyl starch (up to 8 mL/kg and 10 mL/kg, respectively) significantly reduced time to endpoint resuscitation parameters compared with lactated Ringer’s solution (LRS) (up to 90 mL/kg). Centrally mediated hypotension and peripherally mediated bradycardia and vasodilation are possible with infusion rates greater than 1 mL/kg/minute [105]. Extreme caution is used if administering to patients that are severely dehydrated, hyperosmolar, or have little tolerance for rapid intravascular hydrostatic pressure increases (such as those with active hemorrhage or cardiac dysfunction). Isotonic replacement crystalloids (IRC) contain a sodium concentration similar to that of the normal extracellular fluid compartment, making them the ideal crystalloid for the resuscitation and rehydration phase of the fluid therapy plan. Plasmalyte‐A®, Normosol‐R®, and lactated Ringer’s solution contain buffers and are the preferred choice for restoring intravascular volume in most hypovolemic patients (see Table 2.4). The buffer lactate is converted to bicarbonate by the liver and kidney, and is not likely to cause a clinically sustained change in blood lactate [106]. In patients with severe liver dysfunction or neoplasia, prolonged elevations in lactate may occur after lactated Ringer’s administration, but the clinical significance of this is unknown [107–109]. Acetate and gluconate buffers are metabolized to bicarbonate by the liver as well as muscle tissue. Theoretically, the calcium in lactated Ringer’s solution may precipitate the citrate anticoagulant when administered through the same line as blood products, but this has not been shown to be relevant when blood products have been prepared according to blood banking standards [110]. Supplemental electrolytes can be added to isotonic fluids according to patient requirements. As a safeguard in preventing acute hyperkalemia, the rate of potassium administration added to resuscitation fluids should not exceed 0.5 mEq/kg/hour unless carefully monitored. Table 2.4 Resuscitation endpoints targeted with fluid resuscitation in the dog and cat. Normal saline (0.9% sodium chloride) solution is an IRC with a high chloride concentration (154 mmol/L) and low pH (5.0) compared to plasma, and it does not contain additional electrolytes or buffer. This acidifying solution is best used for treating hypochloremic metabolic alkalosis or as a carrier fluid. Under normal conditions, approximately 80% of the crystalloids administered intravenously will filter into the interstitium within an hour, according to normal extracellular TBW distribution. However, IRC can be an effective means of restoring perfusion parameters when the cause of hypovolemia can be rapidly corrected, and the interstitial compartment is capable of handling this additional fluid load. The detrimental effects of rapid, large‐volume crystalloid administration increase when moderate‐to‐severe anemia is present, when increased capillary permeability exists or when the lymphatics cannot manage and the affected organs cannot tolerate additional fluid load (lung, brain, heart). The addition of colloid fluids during resuscitation from hypovolemic shock in these situations becomes important. Colloid fluids are isotonic fluids containing a significant concentration of molecules larger in size than the capillary pore that contribute to COP. Whole blood, plasma, and concentrated albumin have natural colloids in the form of proteins. Hydroxyethyl starches (HES; hetastarch, tetrastarch) are synthetically derived colloids. Table 4.6 and Table 4.7 in Chapter 4 list the natural and synthetic colloids respectively, which are commonly used in the critical small animal ICU patient; dose recommendations are also listed. Blood products are administered when albumin, antithrombin, coagulation factors, platelets, or red blood cells are required. Prior to administering red blood cells, it is ideal to determine the blood type of the patient and to perform a cross‐match in order to administer compatible blood and limit transfusion reactions. In the dog, a DEA 1.1‐negative transfusion is ideal to administer if blood typing or cross‐match is not possible. Since the infusion of incompatible blood products can result in life‐threatening reactions (especially in the cat), determining the blood type and performing a cross‐match are strongly recommended. Unfortunately, catastrophic situations can arise requiring an immediate infusion of blood to save the life of the patient. The use of DEA 1.1‐negative donor blood in the dog, type‐specific blood in the cat (see Chapter 10) or an autologous blood transfusion can minimize the incidence of serious transfusion reactions. Plasma transfusion administration does not require patient blood typing or a cross‐match. An in‐line 18‐micron micropore filter is used during administration of plasma and a 170–210 micron blood administration set for products containing red cells. See Chapters 9 and 10 for additional information on blood products. Human and canine albumin products can be administered to supplement albumin and to maintain COP in the intravascular space. Plasma transfusions have an albumin concentration equal to normal plasma and may not be an effective colloid when used alone. Large volumes may be needed to affect the albumin concentration, which can be cost prohibitive. A 5 g lyophilized canine albumin product (Animal Blood Resources International, Dixon, CA), has been used at a 5% concentration in the treatment of hypotension and hypoalbuminemia in dogs [111]. It is stored in a dehydrated powder form and reconstituted with isotonic saline to a desired concentration. The high concentration of albumin and COP (200 mmHg) in 25% human albumin has the greatest capability of increasing plasma COP and albumin [112–115] but severe and deadly hypersensitivity reactions have been reported in dogs. Additional information regarding the administration of albumin can be found in Chapter 4. Synthetic colloid fluids contain large molecular weight particles that effectively increase COP beyond what can be obtained with blood product infusion alone. They maintain intravascular COP because their average molecular size is too large to pass through the normal capillary pores. A list of synthetic colloids and their characteristics is provided in Table 4.7 (Chapter 4). Synthetic colloids are administered with IRC to restore intravascular volume. Synthetic colloids do not provide albumin, hemoglobin, antithrombin, platelets, or coagulation proteins, but can be administered simultaneously with blood products. They are not used for interstitial volume replacement. Elimination of smaller molecular weight particles is through glomerular filtration. Larger particles are eliminated in bile, stored in tissue, or broken down into smaller particles by the monocyte‐macrophage system and tissue or blood enzymes such as amylase. Hydroxyethyl starch is the parent name of a synthetic polymer of glucose (98% amylopectin), made from a waxy species of either plant starch maize or sorghum. It is a highly branched polysaccharide closely resembling glycogen, formed by the reaction between ethylene oxide and amylopectin in the presence of an alkaline catalyst. The molecular weight and molar substitution can be adjusted by the degree of substitution of hydroxyl groups with hydroxyethyl groups at the C2, C3, and C6 positions on the glucose molecule. The greater the substitution on position C2 in relation to C6 (C2:C6 ratio), the slower the degradation of the molecule by amylase [116]. The number‐averaged molecular weight (Mn) is the arithmetic mean of the molecular weights of the polymers in solution. Weight‐averaged molecular weight (Mw) is the sum of the number of molecules at each number‐averaged molecular weight divided by the total of all molecules. This weight is generally larger when larger polymers are present in solution. There are two types of HES products clinically available in the US at this time: hetastarch and tetrastarch. Hetastarch can be purchased in 0.9% sodium chloride (Hespan™, B Braun Medical, Inc., Bethlehem, PA; Mw of 600 kD and 0.7 degree of substitution) or in LRS (Hextend™, BioTime, Inc., Alameda, CA; a Mw of 670 kD and 0.75 degree of substitution). The electrolyte and buffer compositions of Hextend may reduce the incidence of hyperchloremic acidosis. Hextend also contains 0.45 mmol/L magnesium and 99 mg/dL (0.99%) dextrose. VetStarch™ (Abbott Laboratories, North Chicago, IL) has a Mw of 130 kD and a 0.4 degree of substitution and Tetraspan™ 6% (Braun Medical, Inc.) has a Mw of 130 kD and a 0.42 degree of substitution. Hydroxyethyl starch (HES) 130/0.4 doses have been recommended up to 50 mL/kg in people. Hydroxyethyl starch can affect von Willebrand’s factor, factor VIII function, and platelet function. However, the coagulation effect of HES is no greater than that of saline, and less than that of HTS solutions at equivolume as well as clinically relevant dilutions [117]. While HES administration can provide important and lasting benefits, potential limitations must be recognized. Hydroxyethyl starches will increase coagulation times, particularly when high doses are administered. Literature has reported evidence for an increased risk for acute kidney injury in critically ill people and people with severe sepsis when treated with HES [116,118–120]. This complication has not been identified in dogs or cats receiving 6% hetastarch or 6% tetrastarch. A retrospective study of dogs receiving 10% HES 250/0.5 (pentastarch) has reported an association between the use of 10% pentastarch and adverse outcome [121]. This may be related to the type and concentration of the HES administered. A study of ovine endotoxemic shock found that saline‐based 10% HES 200/0.5 was linked to impaired renal function and more pronounced tubular epithelial injury when compared with 6% HES 130/0.4 and balanced crystalloids [122]. Further research is required to document the incidence and severity of any detrimental responses to 6% hetastarch or tetrastarch in dogs and cats. The fluid resuscitation plan should include the following steps: (1) determine whether there is an intravascular volume deficit affecting perfusion, (2) select fluid(s) specific for the patient, (3) determine resuscitation endpoints, and (4) determine the resuscitation technique to be employed (Box 2.3). Careful patient assessment is essential to detect clues to the underlying cause of the poor perfusion and to identify any important consequences of the inciting disorder. This information will guide the entire resuscitation plan, influencing fluid choice, resuscitation endpoint selection, and, most importantly, the fluid infusion technique.
Fluid balance
Introduction
Diagnostic and monitoring procedures
History and physical examination
Physical and measured parameter
Normal
Compensatory stage of shock
Early decompensatory stage of shock
(middle)
Late decompensatory stage of shock
(terminal)
Level of consciousness
Alert
Normal
Normal to decreased
Decreased to moribund
Heart rate (bpm)
Dogs: 60–120
Cats: 170–200
Dogs: >140
Cats: >170
Dogs: >140
Cats: <180
Dogs: <140
Cats: <160
Mucous membrane color
Pink
Red
Pale
Gray to white
Capillary refill time (seconds)
1–2
<1
>2
>3 to none
Peripheral pulse intensity
Strong
Bounding
Weak
Weak to absent
Rectal temperature °F
(°C)
100–102.5
(37.7–39.2)
100–102.5
(37.7–39.2)
Dogs: low normal
Cats: <98 (<36.7)
Dogs: 98–100
(36.7–37.7)
Cats: <98 (36.7)
Blood pressure
(indirect – mmHg)
Systolic
Mean
90–120
80–100
>100
>80
<100
<80
<80 may not be
<60 detectable
CVP
(cmH2O)
−1 to 5
Variable
<5
Variable; can be high with heart failure
Urine output
mL/kg/h
1–2
Variable
<1
<0.08
Estimated % dehydration
Physical exam findings
0–4
No physical exam changes
4–6
Tacky mucous membranes
6–8
Loss of skin turgor
Dry mucous membranes
Slight hemoconcentration
8–10
Loss of skin turgor
Dry mucous membranes
Retracted globes within orbits
Moderate hemoconcentration
10–12
Persistent skin tent due to complete loss of skin elasticity
Dry mucous membranes
Retracted globes
Dull corneas
Severe hemoconcentration
>12
Persistent skin tent
Dry mucous membranes
Retracted globes
Dull corneas
Signs of perfusion deficits (see Table 2.1)
Extreme hemoconcentration
Point of care testing
Clinicopathological laboratory testing
Diagnostic imaging
Monitoring procedures
Advanced monitoring procedures
Disorders of fluid balance
Intravascular fluid excess
Intravascular fluid deficits
Intravascular fluid deficit resuscitation plan
Fluid choices
Crystalloids
Crystalloid
Tonicity
Osmolarity (mOsm/L)
Na+
(mEq/L)
K+
(mEq/L)
Ca++
(mg/dL)
(mmol/L)
pH
Buffer
Common indications
for selection
Ringer’s lactate
Isotonic
273
130
4
2.7
(0.675)
6.7
lactate
Extracellular volume replacement of hyponatremic patient
Plasmalyte‐A®
Isotonic
294
140
5
0
7.4
acetate, gluconate
Extracellular volume replacement
Normosol‐R®
Isotonic
295
140
5
0
7.4
acetate, gluconate
Extracellular volume replacement
Normal saline (0.9%)
Isotonic
308
154
0
0
5.7
no buffer
Metabolic alkalosis, severe hypochloremia,
hypercalcemia
7% hypertonic saline
Hypertonic
2395
1198
0
0
5.0no buffer
Rapid IV volume resuscitation
0.45% saline with or without 2.5% dextrose
Isotonic then hypotonic (hypotonic without dextrose)
280
77
0
0
4.5
no buffer
Replace solute‐free water deficit
Dextrose (5%) in water
Hypotonic
253
0
0
0
5.0
no buffer
Replace solute‐free water deficit,
drug carrier
Parameter
High normal
Low normal
Level of consciousness
Alert, responsive
Alert, responsive
Heart rate (bpm)
Dog
Cat
80–140
>160
80–140
>160
Mucous membrane color
Pink
Pale pink
Capillary refill time (seconds)
1–2
<2
Peripheral pulse intensity
Strong
Palpable central and peripheral pulses
Rectal temperature
99–101 °F
37.2–38.3 °C
>98 °F
36.6 °C
Indirect blood pressure (mmHg)
Systolic
Mean
90–120
80–100
>90
60–80
Central venous pressure
(cmH2O)
8–10
3–6
Urine output
(mL/kg/h)
1–2
1.0
Lactate
(mmol/L)
<2.0
Declining trend to <2.0
ScvO2
%
>70
>70
Colloids
Blood products
Synthetic colloids
Four‐step intravascular fluid resuscitation plan
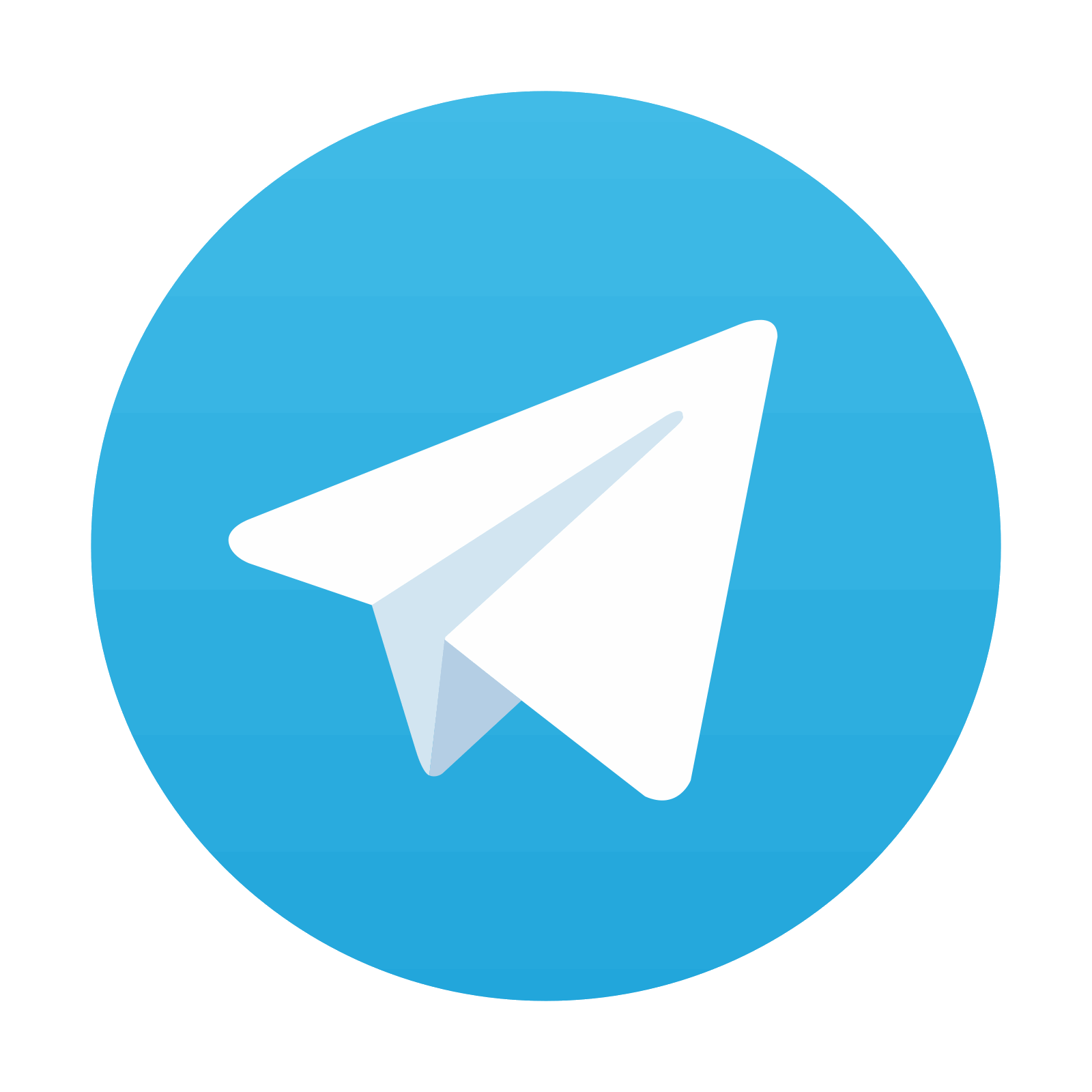
Stay updated, free articles. Join our Telegram channel
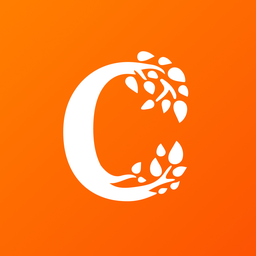
Full access? Get Clinical Tree
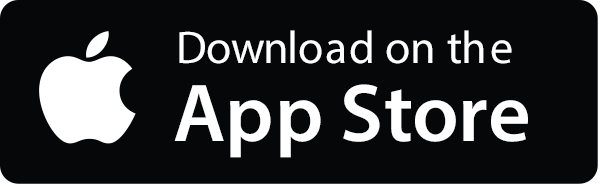
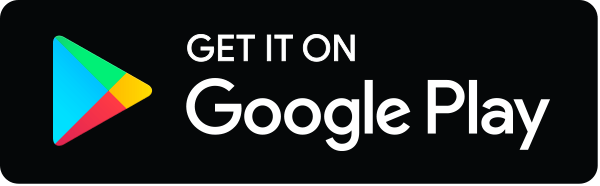