CHAPTER 1 Christine Theoret, DMV, PhD, Diplomate ACVS Prior to undertaking the management of a wound, the underlying biology of wound healing must be understood so that the best approach at the correct time can be selected, and so that problems with healing, if they arise, are recognized. This chapter aims to provide an update on the physiologic, cellular, biochemical, and molecular aspects of wound repair. This chapter is reprinted, in a modified form, from Equine Surgery, 3rd edition, Theoret CL, Wound repair, pp. 44–62, Copyright (2005),1 with permission from Elsevier. A vital trait of living organisms, continually subjected to insults from the environment, is their capacity for self repair. Whether the injury is from surgery or accidental, it generates an attempt by the host to restore continuity to tissue. Two processes are involved in healing: regeneration and repair. Regeneration entails the replacement of damaged tissue with normal cells of the type lost and is only possible in tissues with a sustained population of cells capable of mitosis, such as epithelium, bone, and liver. Conversely, repair is a “stop‐gap” reaction designed to re‐establish the continuity of interrupted tissues with undifferentiated scar tissue. Repair is, therefore, an inferior method of healing, producing scar tissue that is less biologically useful than the tissue it replaced, and that may adversely affect adjacent normal tissues. When complications of wound healing arise, the final result is even worse. Accidental wounds occur commonly in horses and exert a significant welfare concern and financial burden on the equine industry. A large study by the United States Department of Agriculture’s National Animal Health Monitoring System found, in 2006, that injury/wound/trauma was the most common medical condition affecting horses, with a prevalence of 4.7% in equids 6 months of age and older.2 Injury/wound/trauma was the leading cause of death of foals less than 6 months old, accounting for 24% of deaths, while for horses at least 6 months old, it accounted for 16% of deaths and was the leading cause of mortality, after old age.2 A study in Mexico conducted specifically on a population of working equids found a prevalence of 20.6% for cutaneous pathologic conditions; among these, skin wounds (abrasions, lacerations, abscesses) were the most prevalent (6.8%).3 Figures are also concerning in Europe. A study conducted in the UK found that wounds were the most common type of injury reported by horse owners, accounting for roughly half of all injuries occurring over a 12‐month period.4 Another study found that wounds accounted for 21.6% of veterinary treatments of injured polo ponies in the UK.5 Horses in the southern hemisphere do not seem to fare any better: wounds ranked as the third most common medical condition encountered by equine practitioners in Australia and New Zealand and ranked second, after colic, as the most common cause of death or euthanasia.6,7 Finally, skin trauma/wounds are a frequent cause of morbidity in athletic horses. A study in Thoroughbred racehorses has shown that 70% of injuries leading to early retirement are the result of a musculoskeletal injury, of which 7% are associated with wounds or lacerations.8 The objective of repair is to re‐establish an epithelial cover and to recover the integrity, strength, and function of the skin. Partial‐thickness cutaneous wounds (e.g., abrasions and erosions) heal primarily by migration and proliferation of epidermal cells from the remaining underlying epithelium, as well as from the adnexal structures (i.e., hair follicles and sweat and sebaceous glands), with little participation of inflammatory or stromal cells. In contrast, second‐intention repair of full‐thickness cutaneous wounds hinges on four coordinated and interrelated phases (Figure 1.1). Partitioning the process into discrete phases suggests simplicity while, in reality, healing is exquisitely complex. The phases rely on interactions between multiple cellular types, their surrounding matrix, and the soluble mediators that govern the numerous activities required to rebuild the tissue. Moreover, the interactions are not static but rather in a state of constant flux, resulting in a microenvironment that is continually evolving as the wound heals.10 Figure 1.1 Temporal profile of synchronized phases and gain in tensile strength of healing cutaneous wounds. Solid lines show the healing profile of laboratory animals while superimposed shaded areas show the profile of healing full‐thickness wounds on the limb of horses. It should be noted that the timescale is suggestive and depends on the size and extent of the wound. Source: Modified by Marco Langlois (Faculté de médecine vétérinaire, Université de Montréal) from Stashak & Theoret 2014.9 Reproduced with permission of Elsevier. Before veterinarians can positively influence wound healing, they must understand its mechanisms so that they select the appropriate techniques of wound management. In fact, Hippocrates once said, “Healing is a matter of time, but it is sometimes also a matter of opportunity.”11 The skin is the largest organ and serves key functions including physical protection, sensation, temperature regulation, and insulation. It is composed of two compartments – the epidermis and the dermis (Figure 1.2a). In the horse, the epidermis consists of five layers of keratinocytes: the stratum basale, stratum spinosum, stratum granulosum, stratum lucidum, and stratum corneum (Figure 1.2b). Additional epidermal components, referred to as skin appendages, include hair follicles, sweat glands, sebaceous glands, and hooves/nails. Although 90–95% of the cells populating the epidermis are keratinocytes, this compartment also includes melanocytes, Langerhans cells, and Merkel cells. Epidermis is attached to the dermis at the level of the basement membrane, a thin, glycoprotein‐rich layer composed primarily of laminin and type IV collagen. This attachment is through hemidesmosomes, which physically attach the basal cells of the epidermis to the underlying dermis, as well as by vertically oriented type VII collagen anchoring fibrils, which bind the cytoskeleton.12 Figure 1.2 (a) Diagram of a cross‐section of skin, showing the epidermal and dermal compartments. (b) Diagram of the layers of the epidermis of horse skin. Source: Stashak & Theoret 2014.9 Reproduced with permission of Elsevier. The dermal compartment consists of two regions, the papillary dermis and the reticular dermis. This compartment is composed of dense, fibroelastic connective tissue and constitutes the bulk of the skin. The epidermis projects into this underlying connective tissue via extensions known as rete pegs or ridges. A network of collagen fibers provides tensile strength to the dermis, and elastin and glycosaminoglycans (GAGs) ensure resilience. Collagen type I is the major collagen of the dermis (~62%) whereas collagen type III comprises ~15% of the dermis.13 The fibroblast is the principal type of cell found in the dermis; perivascular mast cells and tissue macrophages are also found within the dermis. The connective tissue supports these cells and also a network of nerves, epithelial glands, keratinizing appendages, and a microvascular and lymphatic system. Indeed, only the dermal compartment is vascularized; nutrients reach the epidermis by diffusion. In the horse, the thickness of the skin varies according to body site. For example, in the Dutch warmblood, the skin on the head, neck, and ventral abdomen is relatively thin, measuring between 1.73 mm (±0.16) and 2.03 mm (±0.2) whereas the skin on the limbs is slightly thicker, measuring an average of 2.83 mm (±0.27) for the forelimb and 2.89 mm (±0.24) for the hindlimb, depending on the specific anatomic location (e.g., the skin over the dorsal coffin joint is particularly thick, measuring 4.54 mm [±0.39]).14 The subcutaneous tissue (i.e., tissue just deep to the skin) is also known as the hypodermis or superficial fascia and is not considered part of the skin. It is comprised of loose connective tissue; approximately half of the body’s fat stores are located in this region. The hypodermis anchors the skin to the underlying organs and allows the skin to move relatively freely. It also acts as a shock absorber and insulates the deeper body tissues from heat loss. The first phase of wound healing begins immediately upon injury, is completed within hours, and is dedicated to hemostasis and the formation of a provisional wound matrix. Hemostasis was long considered to be a component of the inflammatory phase, and only recently has its individual significance to wound healing been recognized. Many of the processes that occur during the ensuing phases rely on the normal execution of this short, but critical, initial phase. Wounding traumatizes blood vessels, which results in hemorrhage. The injured endothelial cellular membrane releases phospholipids that are transformed into arachidonic acid and its metabolites that mediate vascular tone and permeability. Peripheral vasoconstriction, lasting 5–10 minutes, limits bleeding but simultaneously starves the surrounding tissues of oxygen and nutrients normally carried by the blood. The resulting transitory hypoxia and increased glycolysis, as well as pH changes, are beneficial because, along with the effects of the original vascular trauma, they enhance the activation, adhesion and aggregation of platelets, thereby initiating the intrinsic coagulation cascade, ultimately leading to the formation of a blood clot that seals the vessel.15 This clot, besides providing a small amount of strength to the wound, has multiple roles. It forms a provisional matrix, rich in fibrin, fibronectin, vitronectin, and thrombospondin, that fills the space created by the wound and serves as a scaffold for migrating cells. Special surface receptors (integrins) on inflammatory and stromal cells recognize binding sites on the proteins within the scaffold, ensuring ingrowth of cells involved in healing. This cellular influx is mediated by chemoattractants released by degranulating platelets, among other cells, present in the scaffold. Indeed, activated platelets are amongst the earliest promoters of inflammation, via the release of potent chemoattractants and mitogens from their storage granules.16 Mediators released not only by platelets but also by mast cells modify vascular tone and permeability, which increase within 5–10 minutes of wounding (apparent clinically as a localized redness, heat and swelling of the wound), and thereby facilitate the aforementioned cellular migration and the diffusion of nutrients and oxygen required to sustain these newly arriving cells. Over time, the surface clot desiccates to form a scab that protects the wound from infection. This scab is, in turn, lysed by plasmin and sloughs, along with dead inflammatory cells and bacteria, as healing proceeds underneath. The inflammatory phase of the wound healing cascade (also referred to clinically as the debridement phase) is activated during the hemostasis/coagulation phase. It can roughly be divided into an early phase, characterized by recruitment of neutrophils, and a late phase, characterized by the appearance and transformation of monocytes. Inflammation prepares the wound for the subsequent phases of healing. It purges the body of alien substances and disposes of dead tissue, while the participating cellular populations liberate mediators to amplify and sustain the events to follow. The intensity of the inflammatory response is strongly correlated to the severity of trauma and determines the extent of scarring. Leukocytes are recruited from blood circulating to the site of injury by the numerous vasoactive mediators and chemoattractants supplied by the coagulation and activated complement pathways, by platelets, by mast cells,10 and by injured or activated stromal cells.17 These signals initiate the processes of rolling, activation, tight adhesion, and, finally, transmigration of inflammatory cells through the microvascular endothelium. Chemoattractants also stimulate the release of enzymes by the activated neutrophils, expediting their penetration through vascular basement membranes. Diapedesis of neutrophils is further facilitated by increased capillary permeability brought about by the release of a spectrum of vasodilatory agents. Cellular influx begins within minutes and the concentration of neutrophils at the wound progressively increases to reach a peak 1–2 days after injury. Neutrophils act as a first line of defense in contaminated wounds by destroying debris and bacteria through phagocytosis and subsequent enzymatic and oxygen‐radical mechanisms. Neutrophil migration and phagocytosis cease when contaminating particles are cleared from the site of injury. Most cells then become entrapped within the clot, which is sloughed during the later phases of repair. The neutrophils remaining within viable tissue die in a few days and are phagocytized by the tissue macrophages or modified wound fibroblasts, marking the termination of the early inflammatory phase of repair.17 Although neutrophils help create a favorable environment within the wound and serve as a source of pro‐inflammatory cytokines, they are not essential to repair of non‐infected wounds. Indeed, a classic series of experiments in the 1970s showed that, as long as conditions were kept sterile, depletion of neutrophils in a guinea pig wound model seemed not to perturb tissue repair.18 More recent knockdown experiments in mice support the depletion studies of the 1970s and go further, showing that repair is even more rapid than in wild‐type sibling mice so long as conditions are sterile.19 The rapid increase in the number of macrophages in inflamed tissue is predominantly caused by the emigration of monocytes from the vasculature, followed by differentiation of the monocytes into macrophages to assist resident tissue macrophages at the wound site for a period of days to weeks. In this manner, the responsive and adaptable pluripotent monocytes can morph into macrophages whose functional properties are determined by the conditions they encounter at the site of mobilization and that change during healing. Macrophages play a central role in all phases of wound healing and orchestrate the overall process. During the early inflammatory phase, macrophages exert pro‐inflammatory functions, such as antigen presentation, phagocytosis, and the production of inflammatory cytokines and growth factors that facilitate wound healing (Figure 1.3). The phenotype of wound macrophages during this phase is probably the classically activated or so‐called “M1 phenotype.” Later, during the proliferative phase of healing, macrophages stimulate proliferation of dermal, endothelial, and epithelial tissue to complete formation of the extracellular matrix (ECM), angiogenesis, and epithelialization. Macrophages can then change the composition of the ECM during the remodeling phase by releasing degradative enzymes (Figure 1.4). This suggests an important role for alternatively activated macrophages (also known as “M2 phenotype”) in this phase of wound healing.20,21 Figure 1.3 Illustration of a full‐thickness cutaneous wound showing the cellular and molecular components present 3 days after injury. FGF, basic fibroblast growth factor; IGF, insulin‐like growth factor; KGF, keratinocyte growth factor; PDGF, platelet‐derived growth factor; TGF, transforming growth factor; VEGF, vascular endothelial growth factor. Source: Singer 1999.17 Reproduced with permission of NEJM. Figure 1.4 Illustration of a full‐thickness cutaneous wound 5 days after injury showing angiogenesis, fibroplasia, and epithelialization. uPA, urokinase‐type plasminogen activator; tPA, tissue‐type plasminogen activator; MMP, matrix metalloproteinase. Source: Singer 1999.17 Reproduced with permission of NEJM. More recent studies using genetically modified neonatal mice have shown that, like neutrophils, macrophages might not be essential for tissue repair.22 Nevertheless, they probably play an important role in the regulation of fibrosis and scarring by degrading matrix.23 In spite of this new, somewhat conflicting evidence, acute inflammation is still considered crucial to the normal repair of wounds in adult mammals exposed to infective agents. When inflammation fails to resolve, however, and a chronic inflammatory response is established, the process can become dysregulated, resulting in pathologic wound repair and the accumulation of permanent fibrotic scar tissue at the site of injury. This fibrosis is characterized by the excessive accumulation of ECM components, including collagens, fibronectin, and hyaluronic acid at the site of injury, leading to a decrease in organ function and, in some cases, organ failure and death. In humans, an estimated 45% of deaths in the western world are now attributed to diseases in which fibrosis plays a major etiologic role.24 One such “fibroproliferative disorder” encountered in full‐thickness cutaneous wounds of the horse and that leads to increased scarring is the development of exuberant granulation tissue (the reader is referred to Chapter 15). After injury, once infection has been countered and repair completed, all the inflammatory cells disperse from the wound. For inflammation to resolve, each of the events that initiated it must be halted or even reversed. Apoptosis, or programmed cellular death, is the universal pathway for eliminating unneeded cells in a phagocytic process that does not elicit additional inflammation.25 This mechanism prevails during all phases of wound repair because each phase relies on rapid increases in specific cellular populations that prepare the wound for repair (inflammatory cells) or deposit new matrices and mature the wound (stromal cells), but then must be eliminated prior to progression to the next phase of repair. Indeed, a mature scar is typically acellular. There are several steps at which the process of resolution could go astray, leading to suppuration, chronic inflammation (Figure 1.5), and/or excessive fibrosis. Figure 1.5 This metatarsal wound failed to heal for 7 months as a result of chronic low‐grade inflammation due to exposure as well as superficial and deep infection. The wound in fact became larger rather than smaller, illustrating suspension of the healing process. Courtesy of Dr. Derek Knottenbelt. The main objective of the proliferative phase (also referred to clinically as the repair phase) is to achieve protection of the wound’s surface via the formation of granulation tissue and a new epithelial cover and to restore the vascular network to nourish the new tissues. The proliferative phase of repair comes about as inflammation subsides and is characterized by the appearance of red, fleshy granulation tissue, which ultimately fills the defect. Although the earliest part of this phase is very active on a cellular level, this activity does not immediately translate into a gain in the wound’s strength. Indeed, during the first 3–5 days following injury, fibroblasts and endothelial and epithelial cells rapidly invade the wound in preparation for synthesis and maturation of the matrix or for wound coverage; however, these latter reinforcing mechanisms lag somewhat. Granulation tissue is formed by three elements that simultaneously move into the defect created by the wound: macrophages, which debride and produce mediators, such as cytokines and growth factors, that stimulate angiogenesis and fibroplasia; fibroblasts, which proliferate and synthesize new components of the ECM; and new blood vessels, which carry oxygen and nutrients necessary for the metabolism and growth of cells, and confer to the granulation tissue its characteristic red, granular appearance. This stroma, rich in fibronectin and hyaluronan, replaces the fibrin clot to provide a physical barrier to infection and, importantly, provides a surface across which cells can migrate. A number of matrix molecules as well as cytokines and growth factors released by inflammatory cells are believed to stimulate fibroblasts from adjacent uninjured dermis and subcutaneous tissue to proliferate and express integrin receptors to assist their migration into the defect. Integrins are transmembrane proteins that act as the major cell‐surface receptors for ECM molecules and thus mediate interactions between cells and their environment. They are particularly critical to the migratory movements exhibited by cells involved in wound healing, such as epithelial and endothelial cells and fibroblasts.26 Migration of fibroblasts immediately precedes advancing capillary endothelial buds but follows macrophages that have cleared a path by phagocytizing debris. Fibroblasts themselves also possess an active proteolytic system, comprising proteinases, such as plasminogen activator (PA), various collagenases, gelatinase, and stromelysin,27 to aid their migration into the fibrin blood clot. After fibroblasts have arrived within the defect created by the wound, they proliferate then switch their function to protein synthesis and commence to gradually replace the provisional matrix with a collagen‐rich one, probably under the influence of various cytokines and growth factors. As the wound matures, the ratio of type I (mature) to type III (immature) collagen markedly increases; proteoglycans also become abundant within the mature matrix. The greatest rate of accumulation of connective tissue within the wound occurs 7–14 days after injury, at least in the laboratory rodent, which translates into the period of most rapid gain in tensile strength (see Figure 1.1). Thereafter, the collagen content within the wound levels off as fibroblasts down‐regulate their synthetic activities; this corresponds to a much slower gain in tensile strength as the wound remodels. The fibroblast‐rich granulation tissue is subsequently replaced by relatively avascular and acellular scar tissue, as the capillaries within the wound regress and fibroblasts either undergo apoptosis28 or acquire characteristics of smooth muscle and transform into myofibroblasts that participate in wound contraction. The latter phenomena are regulated by the physiologic needs and/or the microenvironmental stimuli present at the wound. It appears that if the signal to down‐regulate fibroblast activity is delayed beyond a specific time point, apoptosis is permanently impaired, ultimately leading to an imbalance between collagen synthesis and degradation29 and the formation of excessive scar tissue. Besides initiating the inflammatory response through interactions with leukocytes, microvascular endothelial cells play a key role in the proliferative phase of repair. The formation of new capillaries from pre‐existing ones (angiogenesis) is necessary to restore oxygenation and to provide required nutrients to the granulation tissue newly formed within the wound. Angiogenesis, which occurs in response to tissue injury and hypoxia, is a complex and dynamic process mediated by diverse soluble factors provided by the serum and the surrounding ECM. These factors are, in particular, angiogenic inducers, including growth factors [most notably vascular endothelial growth factor (VEGF), fibroblast growth factor (FGF)‐2, platelet‐derived growth factor (PDGF), and members of the transforming growth factor (TGF)‐β family],30 chemokines and angiogenic enzymes (notably the serine proteinase thrombin), endothelial‐specific receptors, and adhesion molecules, such as integrins,31 many of which are released during the inflammatory phase of repair. Construction of a vascular network requires sequential steps that include augmented microvascular permeability, the release of proteinases from activated endothelial cells with subsequent local degradation of the basement membrane surrounding the existing vessel, migration and sprouting of endothelial cells into the interstitial space, endothelial cellular proliferation and differentiation into mature blood vessels (i.e., arterioles and venules), eventually followed by regression and involution of the newly formed vasculature as tissue remodels.32 Angiogenesis depends not only on the cells and cytokines present, but also on the production and organization of components of the ECM that provide a scaffold through which endothelial cells can migrate and a reservoir and modulator for growth factors. Thus, endothelial cells at the tip of capillaries begin their migration into the wound in response to angiogenic stimuli and the absence of neighboring cells on the second day after injury. Cytoplasmic pseudopodia extend through fragmented basement membranes; subsequently, the entire endothelial cell migrates into the perivascular space. Cells remaining in the parent vessel near the tip of the angiogenic sprouts begin to proliferate, providing a continuous source of microvascular endothelial cells for angiogenesis. A new capillary sprout has no lumen when it first develops; after it fuses with a neighboring sprout to form an arcade, it canalizes, allowing erythrocytes to pass into and through it. Formation of a lumen probably involves the joining of plasma membranes of individual and/or adjacent cells, as well as extensive intracellular vacuolization followed by fusion of the vacuoles to form “ring cells,” which ultimately fuse to form seamless capillaries. Capillaries then become stable as endothelial cells interact with the new basement membrane within 24 hours of new vessel formation and via the recruitment of pericytes and smooth muscle cells. In healing wounds, this vigorous angiogenic response results in a density of vessels that far exceeds that of capillaries in normal, uninjured tissue33 and provides the granulation tissue with its red, granular appearance. After the stroma has been completely reconstituted, a rich vascular supply to the wound is no longer needed. Pro‐angiogenic stimuli are down‐regulated and/or the local concentration of anti‐angiogenic factors (thrombospondin, interferon gamma‐induced protein 10/CXC motif chemokine 10, and Sprouty‐2)34,35 increases, and most of the recently formed capillary network quickly involutes through the activity of matrix metalloproteinases (MMPs),36 in particular MMP‐1 and MMP‐10,37 and selective apoptosis of endothelial cells. The color of the wound pales as the rich capillary bed disappears from the granulation tissue. Epithelialization is defined as the process of covering denuded epithelial surfaces and is essential for successful closure of the wound. In addition to the aforementioned hemostatic activities that establish a temporary barrier, the residual epithelium beneath the clot moves centripetally to participate in closure of the wound. Even though epithelial migration commences 24–48 hours after wounding, the characteristic pink rim of new epithelium (Figure 1.6) is not macroscopically visible until some time later. This “lag” varies according to the species of animal and the site, size, and substrate of the wound. For example, epithelialization is accelerated in a partial‐thickness wound because migrating cells arise not only from the residual epithelium at the wound’s periphery but also from remaining epidermal appendages. Furthermore, the basement membrane is intact in this type of injury, precluding a lengthy regeneration. On the other hand, during second‐intention healing of a full‐thickness wound, epithelialization cannot proceed until a bed of granulation tissue has formed. In full‐thickness, 7–9 cm2 wounds created experimentally in horses and ponies and left to heal by second intention, after an initial lag phase, epithelialization progressed over the wound surface at a rate of 0.63 mm/week for metatarsal wounds in ponies, 0.48 mm/week for metatarsal wounds in horses, 0.75 mm/week for buttock wounds in ponies, and 0.62 mm/week for buttock wounds in horses between the third and the seventh week of healing.38 Figure 1.6 Large full‐thickness metatarsal wound that healed partially by second intention and was subsequently grafted successfully. The wound showed excellent epithelialization from the healing margin of the wound. The healthy epithelial tissue is characterized by an area of hyperemia adjacent to it. Courtesy of Dr. Derek Knottenbelt. The regenerative capacity of the epidermis relies on keratinocyte stem cells (KSC) that reside within specific microenvironments referred to as stem cell niches. The following three niches have been identified: the bulge of the hair follicle (HF), the base of the sebaceous gland, and the basal layer of the interfollicular epidermis (IFE).39 In response to epidermal injury, the HF and IFE niches participate in epithelialization of the defect.40 To close the defect in the epidermis, keratinocytes at the wound’s edge must first loosen their adhesions to each other (desmosomes) and to the basal lamina (hemidesmosomes) and develop the flexibility required to migrate over the new matrix. Numerous regulators play a critical role in modulating the proliferation and migration of keratinocytes during epithelialization; these include chemokines, cytokines, integrins, keratins, ECM molecules, and MMPs, among others. A discussion of these is beyond the scope of the text; the reader is referred to a recent review of the subject.41 Additionally, various degradative enzymes necessary for the proteolysis of components of the ECM are up‐regulated within cells at the leading edge of neo‐epithelium, facilitating ingestion of the clot and debris found along the migratory route. This route is determined by the array of integrin receptors for various ECM proteins, expressed on the surface of migrating epithelial cells. Once the surface of the wound is covered by epithelial cells contacting one another, further migration is inhibited by the expression, within the ECM, of laminin, a major factor responsible for adhesion of epithelial cells. Although initial cellular migration does not require an increase in cellular multiplication, basal keratinocytes at the wound’s margin do begin to proliferate 1–2 days after injury to replenish the migratory front; this corresponds histologically to epithelial hyperplasia (Figure 1.7). The new cells leap‐frog over those at the wound margin and adhere to the substratum, only to be replaced in turn by other cells coming from above and behind. The newly adherent monolayer subsequently restratifies to restore the original multi‐layered epidermis. Figure 1.7 Photomicrograph of a wound edge sample of tissue. Normal unwounded skin to the left demonstrating epidermal appendages (h, hair follicles); new hyperplastic epithelium (EH) to the right, overlying granulation tissue bed. Source: Theoret 2004.42 Reproduced with permission of Elsevier. In full‐thickness wounds healing by second intention, such as those commonly managed in equine practice, provisional matrix is eventually replaced by a mature basement membrane. Repairing epidermis reassembles its constituents from the margin towards the center of the wound.17 Epidermal cells then revert to a quiescent phenotype and become attached to this new basement membrane through hemidesmosomes and to the underlying neodermis through type VII collagen fibrils. This particular aspect of epithelialization is time consuming, occurring long after total coverage of the wound by epithelium is apparent, which may explain the continued fragility of neoepidermis for extended periods after repair is macroscopically complete. This is particularly evident in large wounds of the limb, where new epidermis is often thin and easily traumatized (Figure 1.8). Figure 1.8 (a) A 5‐day‐old, full‐thickness, experimentally created wound over the dorsal surface of the fetlock. Granulation tissue is beginning to fill the wound. (b) The same wound, 75 days after creation. Neoepidermis is thin, dry, and hairless, and could be easily traumatized. Courtesy of Dr. Ted Stashak. Mature ECM is a non‐cellular scaffold composed of proteins, glycosaminoglycans, polysaccharides, and water, that facilitates bidirectional communication between cells and their biochemical/biophysical environment.43 During the remodeling phase (occurring from 3 weeks to up to 1 year after injury), the components of the ECM undergo certain changes to ensure strength, integrity, and function of the replacement tissue. In addition to epithelialization, contraction contributes to the successful closure of full‐thickness wounds. Contraction is defined as a process whereby both dermis and epidermis bordering a full‐thickness skin deficit are drawn from all sides centripetally over the exposed wound.44 This usually occurs during the second week after injury. Wound contraction not only accelerates closure, it also enhances the cosmetic appearance and strength of the scar because proportionally less area of the wound must be covered by newly formed epithelium of inferior quality, which is fragile and lacks normal nervous, glandular, follicular, and vascular components (Figure 1.8b). For this reason, a high degree of contraction is a desired feature of wound repair, at least in the horse. Wound contraction is thought to result from a combination of tractional forces generated by migrating fibroblasts and the action of a specialized fibroblast phenotype, the myofibroblast. As fibroblasts migrate into the provisional matrix of the wound under the influence of various cytokines, tension within the wound reaches the threshold required, along with the action of TGF‐β1 and the ED‐A splice variant of fibronectin,45 to trigger fibroblasts to differentiate into myofibroblasts.46 The latter are the most abundant cellular elements of healthy granulation tissue and are aligned within the wound along the lines of contraction. The most striking feature of the myofibroblast is a well‐developed alpha smooth muscle actin (α‐SMA) microfilamentous system, arranged parallel to the cell’s long axis and in continuity with the components of the ECM via various integrins. In addition to these cell–substratum links, intercellular connections, such as gap junctions and hemidesmosomes, ensure that neighboring cells exert tension on one another. Wound contraction is divided into three phases. An initial lag phase (wherein skin edges retract, and the area of the wound increases temporarily for 1–2 weeks, depending on its anatomic location) occurs prior to substantial fibroblastic invasion of the wound, as a prerequisite for contraction. Subsequently, a period of rapid contraction is followed by one of slow contraction as the wound approaches complete closure. The number of myofibroblasts found in a wound appears proportional to the need for contraction, and thus, as repair progresses and the rate of contraction slows, this number decreases accordingly. During wound contraction, the surrounding skin stretches by intussusceptive growth, and the wound takes on a stellate appearance. Contraction ceases in response to one of three events: the wound’s edges meet, causing contact inhibition to halt the processes of epithelialization and contraction; tension in the surrounding skin becomes equal to or greater than the contractile force generated by the α‐SMA of the myofibroblasts; or, in the case of chronic wounds, a low number of myofibroblasts in the granulation tissue may result in failure of the wound to contract, despite laxity in the surrounding skin. In the latter case, the granulation tissue is pale and consists primarily of collagen and ground substance. Wound contraction is greater in regions of the body with loose skin than in regions where skin is under tension, such as the distal extremity of the equine limb. Although the shape of the wound has been speculated to influence the process of contraction, this does not appear relevant in wounds of the distal limb where skin is tightly stretched and not easily moved.47 Wound contraction was measured in full‐thickness, 7–9 cm2 experimental wounds left to heal by second intention on the limbs and hindquarters of horses and ponies. The wounds were bandaged for the first 3 weeks then left unbandaged. Following an initial lag phase of 1 week, wound contraction became apparent in buttock wounds of horses and ponies and in metatarsal wounds of ponies. The percentage decrease in wound surface area attributable to wound contraction between the second and the fourth week of healing was 47% for the body wounds of ponies vs. 35% for the limb wounds of ponies, and 38% for the body wounds of horses vs. 0% for the limb wounds of horses. After week 4, the rate of wound contraction slowed to less than 5% per week for these wounds, up to complete healing. The metatarsal wounds of horses showed a different pattern: the lag phase of healing lasted 4 weeks and this was followed by an average rate of contraction that did not exceed 2.5% per week.38 As contraction concludes, myofibroblasts disappear, either by reverting to a quiescent fibroblastic phenotype or by apoptosis,28 primarily in response to reduced tension within the ECM.48 The myofibroblast persists in fibrotic lesions where it may be involved in accumulation of more ECM and pathologic contracture, a condition leading to substantial morbidity, particularly when it involves joints or orifices, but rarely encountered in the horse. The conversion of ECM from granulation to scar tissue constitutes the final phase of wound repair, also referred to as maturation, and consists of synthesis of connective tissue, lysis, and remodeling. Proteoglycans replace hyaluronan during the second week of repair, support the deposition and aggregation of collagen fibers, and make the mature matrix more resilient. Collagen macromolecules provide the tensile strength to the wound as their deposition peaks within the initial week, when healing occurs by first intention, and between 7 and 14 days, when healing occurs by second intention, in the laboratory rodent. Although this corresponds to the period of most rapid gain in strength, only 20% of the final strength of the skin wound is achieved within the first 3 weeks of repair. At this time, collagen synthesis is balanced by collagen lysis, which normally prevents accumulation of excessive amounts of collagen and formation of a pathologic scar. The balance between synthesis and degradation determines the overall strength of a healing wound at a particular time. The first newly deposited collagen tends to be oriented randomly and, therefore, provides little tensile strength, whereas during remodeling, the fibers reform along lines of stress and, therefore, resist dehiscence more effectively. Cross‐linking of the later‐formed collagen is also more effective, but never occurs to the same extent as in the original tissue. The new collagen weaves into that which pre‐existed and also appears to bond to the ends of old collagen fibers. These welds are points of weakness, which may rupture when stressed. Remodeling of ECM within a wound depends on the presence of various proteolytic enzymes (proteinases) released from inflammatory and mesenchymal cells, such as MMPs and serine and cysteine proteinases (cathepsins). Those of the MMP family are collectively capable of degrading virtually all components of the ECM. Although MMPs are not constitutively expressed in skin, up‐regulation occurs whenever proteolysis is required, such as during cellular migration and remodeling of the matrix. Inactive precursors of the MMPs (pro MMPs) are cleaved in the extracellular space by proteinases, such as plasmin and trypsin, left over from the inflammatory and proliferative phases, but also by other MMPs. To date, two dozen different MMPs, all distinct gene products, have been characterized.27 Based on domain organization and preference for substrate, MMPs may be divided into the following groups: 1 – collagenases; 2 – gelatinases; 3 – stromelysins; 4 – matrilysins; 5 – metalloelastases; 6 – membrane‐type MMPs; 7 – other MMPs.49 Although the major function of most MMPs is probably to process bioactive molecules, such as chemokines and cytokines, as well as their respective receptors, their ability to degrade ECM proteins, as demonstrated by some members of the MMP family (MMP1, MMP3, MMP13, and MMP14 are capable of cleaving collagen; MMP7 can process syndecan‐1 and elastin), suggests they have a role in remodeling during wound healing.27 Comprehensive lists of MMPs, including their physiologic and in vitro substrates, can be found in proteinase databases.50,51 Homeostasis between collagen synthesis and degradation during the remodeling phase depends on the simultaneous presence of MMPs and non‐specific inhibitors, such as α2‐macroglobulin and α1‐antiprotease, as well as natural specific inhibitors, the tissue inhibitors of metalloproteinases (TIMPs). TIMPs are a gene family of four structurally related members, TIMP‐1 through ‐4, that inhibit conversion of MMPs from a zymogen to an activated state and that irreversibly bind to the catalytic site of active MMPs.49 Under most circumstances, an imbalance between MMPs and TIMPs leads to abnormal resolution and delayed repair. Indeed, although the presence of MMPs is essential for the wound to mature, it may also be responsible for the inability of chronic wounds to heal. For example, fluid found in chronic wounds is characterized by elevated concentrations of proteinases, particularly MMP‐9 and serine proteinases, which lead to excessive degradation of proteins and the inactivation of critical growth factors. Chronic wounds also contain reduced concentrations of TIMPs, in particular TIMP‐1.52 Wound remodeling continues for up to 2 years, during which time there is no net increase in collagen content, but instead, the collagen fibers rearrange into a more organized lattice‐like structure, under the influence of local mechanical factors, progressively increasing the tensile strength of scar tissue. The majority of type III collagen fibers laid down early in healing are replaced by collagen type I, fibers become increasingly cross‐linked, and the normal skin ratio of 4:1 type I to type III collagen is re‐established. Glycosaminoglycans are steadily degraded until they reach concentrations found in normal dermis. The duration of the maturation phase depends on a variety of factors, including the patient’s genetic makeup, age, location of the wound, type of injury, and duration of inflammation. At maximum strength, cutaneous wounds in mice remain 15–20% weaker than the normal surrounding tissue,53 but a study in horses showed that cutaneous wounds, fully healed by second intention, withstood a maximum breaking load equivalent to only 60% of the breaking force of normal, intact skin.54 Importantly, skin appendages are usually not regenerated after wounding, resulting in a loss of other functions of skin.
Physiology of Wound Healing
Summary
Introduction
Skin anatomy
Phases of wound repair
Hemostasis/coagulation
Inflammation
Cellular proliferation
Fibroplasia
Angiogenesis
Epithelialization
Matrix synthesis and remodeling (also referred to clinically as the maturation phase)
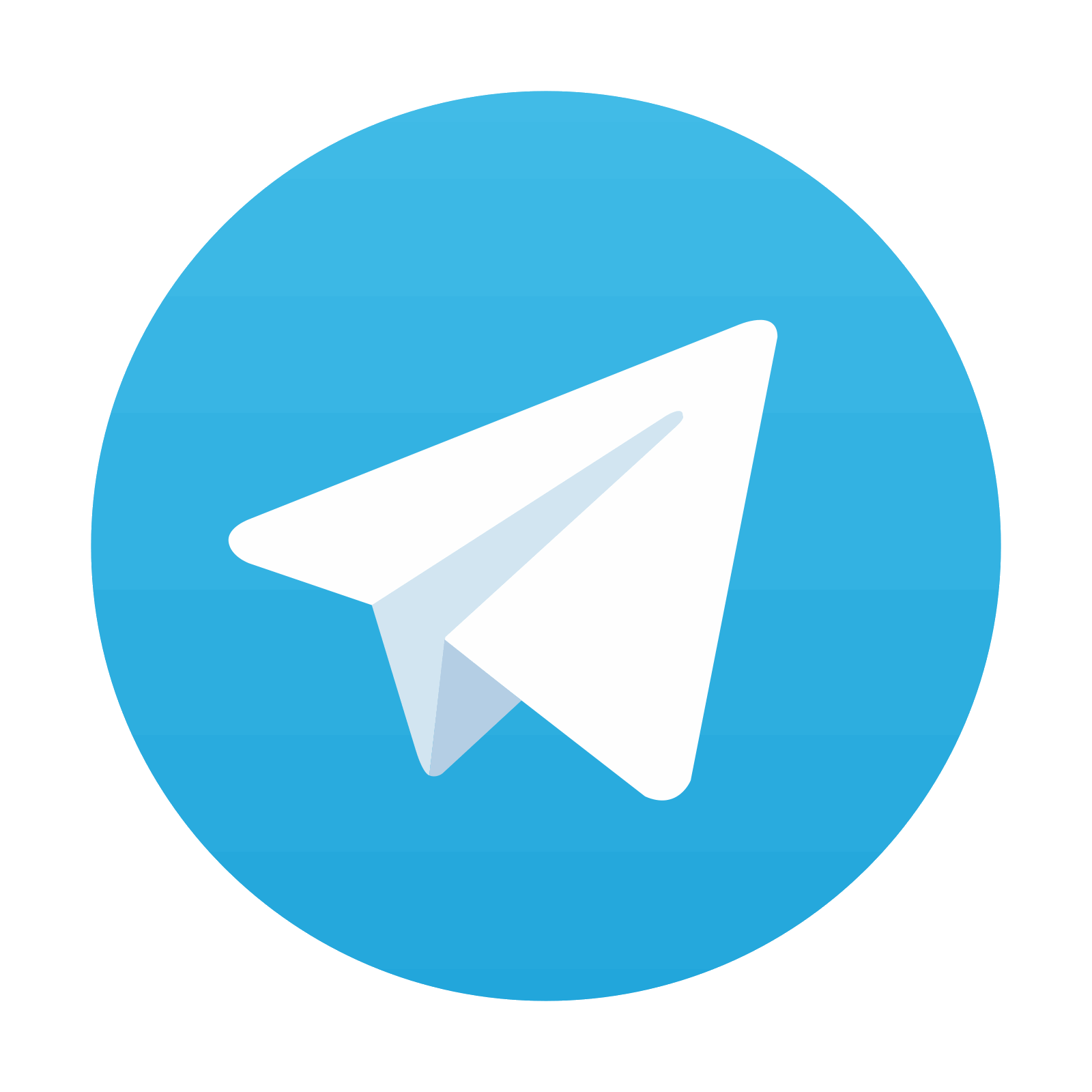
Stay updated, free articles. Join our Telegram channel
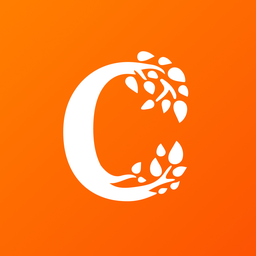
Full access? Get Clinical Tree
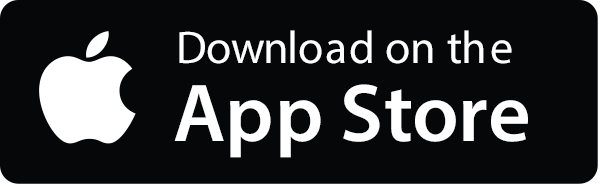
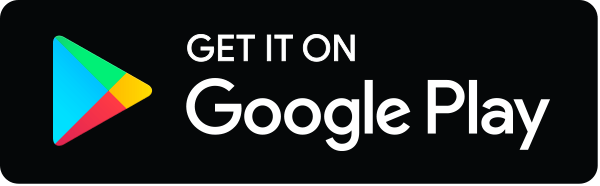